Accessibility Links
- Skip to content
- Skip to search IOPscience
- Skip to Journals list
- Accessibility help
- Accessibility Help
Click here to close this panel.

The American Astronomical Society (AAS) , established in 1899 and based in Washington, DC, is the major organization of professional astronomers in North America. Its membership of about 7,000 individuals also includes physicists, mathematicians, geologists, engineers, and others whose research and educational interests lie within the broad spectrum of subjects comprising contemporary astronomy. The mission of the AAS is to enhance and share humanity's scientific understanding of the universe.

The Institute of Physics (IOP) is a leading scientific society promoting physics and bringing physicists together for the benefit of all. It has a worldwide membership of around 50 000 comprising physicists from all sectors, as well as those with an interest in physics. It works to advance physics research, application and education; and engages with policy makers and the public to develop awareness and understanding of physics. Its publishing company, IOP Publishing, is a world leader in professional scientific communications.
A publishing partnership

High-resolution UV/Optical/IR Imaging of Jupiter in 2016–2019
Michael H. Wong 1 , Amy A. Simon 2 , Joshua W. Tollefson 1,3 , Imke de Pater 1,3 , Megan N. Barnett 4 , Andrew I. Hsu 3 , Andrew W. Stephens 5 , Glenn S. Orton 6 , Scott W. Fleming 7 , Charles Goullaud 3 , William Januszewski 7 , Anthony Roman 7 , Gordon L. Bjoraker 8 , Sushil K. Atreya 9 , Alberto Adriani 10 , and Leigh N. Fletcher 11
Published 2020 April 1 • © 2020. The Author(s). Published by the American Astronomical Society. The Astrophysical Journal Supplement Series , Volume 247 , Number 2 Citation Michael H. Wong et al 2020 ApJS 247 58 DOI 10.3847/1538-4365/ab775f
You need an eReader or compatible software to experience the benefits of the ePub3 file format .
Article metrics
23757 Total downloads
Share this article
Author affiliations.
1 Center for Integrative Planetary Science, University of California, Berkeley, CA 94720, USA
2 Solar System Exploration Division, NASA Goddard Space Flight Center, Greenbelt, MD 20771, USA
3 Astronomy Department, University of California, Berkeley, CA 94720, USA
4 Department of Geophysical Sciences, University of Chicago, Chicago, IL 60637, USA
5 Gemini Observatory North, NSF's National Optical-Infrared Astronomy Research Laboratory, Hilo, HI 96720, USA
6 NASA Jet Propulsion Laboratory, California Institute of Technology, Pasadena, CA 91109, USA
7 Space Telescope Science Institute, Baltimore, MD 21218, USA
8 Code 693, NASA Goddard Space Flight Center, Greenbelt, MD 20771, USA
9 Deptartment of Climate and Space Sciences and Engineering, University of Michigan, Ann Arbor, MI 48109, USA
10 INAF—Istituto di Astrofisica e Planetologia Spaziali, 00133 Roma, Italy
11 School of Physics and Astronomy, University of Leicester, Leicester LE1 7RH, UK
Michael H. Wong https://orcid.org/0000-0003-2804-5086
Amy A. Simon https://orcid.org/0000-0003-4641-6186
Joshua W. Tollefson https://orcid.org/0000-0003-2344-634X
Imke de Pater https://orcid.org/0000-0002-4278-3168
Megan N. Barnett https://orcid.org/0000-0003-4804-577X
Andrew I. Hsu https://orcid.org/0000-0002-6190-9336
Andrew W. Stephens https://orcid.org/0000-0002-4434-2307
Glenn S. Orton https://orcid.org/0000-0001-7871-2823
Scott W. Fleming https://orcid.org/0000-0003-0556-027X
William Januszewski https://orcid.org/0000-0002-3545-1157
Anthony Roman https://orcid.org/0000-0001-5040-8520
Gordon L. Bjoraker https://orcid.org/0000-0002-9679-4153
Alberto Adriani https://orcid.org/0000-0003-4998-8008
Leigh N. Fletcher https://orcid.org/0000-0001-5834-9588
- Received 2019 August 31
- Revised 2020 February 6
- Accepted 2020 February 8
- Published 2020 April 1
Jupiter ; Planetary atmospheres ; Time series analysis ; Direct imaging ; Seasonal phenomena ; Astrophysical fluid dynamics ; Hubble Space Telescope ; Ground-based astronomy ; Atmospheric variability ; Clouds
Receive alerts on all new research papers in American Astronomical Society ( A A S ) journals as soon as they are published. Select your desired journals and corridors below. You will need to select a minimum of one corridor.
Please note, The Planetary Science Journal (PSJ) does not currently use the corridors.
What are corridors? opens in new tab
Imaging observations of Jupiter with high spatial resolution were acquired beginning in 2016, with a cadence of 53 days to coincide with atmospheric observations of the Juno spacecraft during each perijove pass. The Wide Field Camera 3 (WFC3) aboard the Hubble Space Telescope (HST) collected Jupiter images from 236 to 925 nm in 14 filters. The Near-Infrared Imager (NIRI) at Gemini North imaged Jovian thermal emission using a lucky-imaging approach (co-adding the sharpest frames taken from a sequence of short exposures), using the M ' filter at 4.7 μ m. We discuss the data acquisition and processing and an archive collection that contains the processed WFC3 and NIRI data (doi: 10.17909/T94T1H ). Zonal winds remain steady over time at most latitudes, but significant evolution of the wind profile near 24°N in 2016 and near 15°S in 2017 was linked with convective superstorm eruptions. Persistent mesoscale waves were seen throughout the 2016–2019 period. We link groups of lightning flashes observed by the Juno team with water clouds in a large convective plume near 15°S and in cyclones near 35°N–55°N. Thermal infrared maps at the 10.8 micron wavelength obtained at the Very Large Telescope show consistent high brightness temperature anomalies, despite a diversity of aerosol properties seen in the HST data. Both WFC3 and NIRI imaging reveal depleted aerosols consistent with downwelling around the periphery of the 15°S storm, which was also observed by the Atacama Large Millimeter/submillimeter Array. NIRI imaging of the Great Red Spot shows that locally reduced cloud opacity is responsible for dark features within the vortex. The HST data maps multiple concentric polar hoods of high-latitude hazes.
Export citation and abstract BibTeX RIS
- NASA ADS Record opens in new tab
- About Related Links
Original content from this work may be used under the terms of the Creative Commons Attribution 4.0 licence . Any further distribution of this work must maintain attribution to the author(s) and the title of the work, journal citation and DOI.
1. Introduction
The era of high-resolution Jupiter imaging at visible wavelengths began in space, with the Pioneer and Voyager spacecraft flybys (Fountain et al. 1974 ; Smith et al. 1979 ). These missions gave the first looks at discrete features like convective plumes and the first accurate measurements of the zonal winds (or differential rotation). The Hubble Space Telescope (HST) has continued to image the planet at high resolution, as have the Galileo and Juno missions to Jupiter, and flybys from missions like Cassini and New Horizons. Although velocities have been measured in specific locations using Galileo and New Horizons data (Gierasch et al. 2000 ; Hueso et al. 2009 ), only Voyager, HST, and Cassini have done significant time-series imaging capable of measuring the dynamics of jets, waves, and vortices on a global scale (Limaye 1986 ; Simon-Miller et al. 2012 ). Both manual tracking (Mitchell et al. 1981 ) and automated correlation (e.g., Choi et al. 2007 ; Asay-Davis et al. 2009 ) methods have been used to measure velocities from image sequences.
At longer wavelengths near 5 μ m, Jupiter has weak molecular absorption and, thus, emits thermal radiation from deeper levels of 4–7 bar (Bjoraker et al. 1986 ). Like a jack-o'-lantern, Jupiter's appearance is marked by bright "hot spots" that are free from overlying cloud opacity. The inhomogeneous pattern of 5 μ m emission is primarily observed from ground-based facilities (Westphal 1969 ; Harrington et al. 1996 ; Ortiz et al. 1998 ), since the terrestrial atmosphere has good transparency in the infrared M -band (Tokunaga 2000 ), and large telescopes can provide images with excellent angular resolution, particularly when improved with an adaptive optics approach (de Pater et al. 2010 , 2011 ) or a lucky-imaging approach (Fletcher et al. 2018 ), where many short exposures are taken and the sharpest frames are co-added. NASA's giant planet flagship orbiters, Galileo and Cassini, carried imaging spectrometers that covered the 5 μ m range (Carlson et al. 1992 ; Miller et al. 1996 ), and Juno's JIRAM instrument has produced low-resolution spectra and stunning images of Jupiter's atmosphere, particularly in polar regions (Sindoni et al. 2017 ; Adriani et al. 2018b ).
During each spacecraft pass, Juno dips down to 3400–8000 km above the cloud tops at closest approach, and these "perijove" (or PJ) encounters occur once every 53 days in Juno's highly eccentric ( e = 0.98) orbit (Bolton et al. 2017 ). Juno's MWR instrument (Janssen et al. 2017 ) has produced remarkable new observations of ammonia opacity and lightning sferics during perijove passes (Li et al. 2017 ; Brown et al. 2018 ). Like cloud opacity, both ammonia mixing ratio (Gierasch et al. 1986 ; Achterberg et al. 2006 ) and lightning flashes are tracers of dynamics: upwelling air tends to have more cloud condensation, higher volatile mixing ratios, and even lightning in the case of moist convection. Downwelling air tends to be depleted in condensable volatiles relative to the surroundings, and depleted in cloud opacity as well.
This paper reports on a sequence of high-resolution imaging data, covering the 250–900 nm range with multiple HST/UVIS filters and the 4.7 μ m wavelength with Gemini/Near-Infrared Imager (NIRI). The core motivation of the data set is to provide consistent context imaging for Juno passes. Some early elements of the data set depart from the regular 53 day cadence because some ground-based observations could not be re-planned to adjust to changes in the Juno trajectory plan (Bolton et al. 2017 ). This paper provides a complete description of the data set, which is available in raw and processed forms online, in order to facilitate future research. Sections 2 and 3 describe the acquisition and processing of the HST and Gemini components of the data set, Section 4 describes the high-level science products (HLSPs) produced from the observations and available online, and Section 5 gives an overview of science results to date. This paper covers data collected in the 2016–2019 time period. More observations are planned and/or proposed for future dates throughout the remainder of the Juno mission.
2. HST WFC3 Imaging Data
We use the UVIS channel of the WFC3 instrument to obtain high-resolution imaging of Jupiter in the UV–NIR range. A description of the instrument, including filter central wavelengths and bandpasses, is given by Dressel ( 2019 ). Filters used for the Jupiter observations reported here are listed in Table 1 . Several specific observing modes, settings, and best practices are commonly used for Jupiter and other planetary observations. Details of these settings have been omitted from prior publications, but we describe them in Sections 2.1 – 2.7 for completeness, for potential benefit to other observers, and to ensure that data products in the MAST archive (Section 4 ) are fully described. Readers who are uninterested in the details of planning HST observations might skip to Section 2.8 , which discusses the temporal sampling within the data set.
Table 1. WFC3/UVIS Filters Used in Relevant Jupiter Programs
Filter | . (s) | S/N | Programs | Objectives |
---|---|---|---|---|
F225W | 40 | 85 | WFCJ, VLA | Haze structure/distribution |
F275W | 20 | 125 | WFCJ, OPAL, VLA | Haze structure/distribution |
F343N | 6 | 160 | WFCJ, OPAL, VLA | Haze distribution/composition |
F395N | 9 | 155 | WFCJ, OPAL, VLA | Haze distribution/composition |
F467M | 1.2 | 170 | OPAL | Haze distribution/composition |
F502N | 4 | 170 | WFCJ, OPAL, VLA | Haze distribution/composition |
F547M | 0.48 | 200 | OPAL | Haze distribution/composition |
F631N | 4 | 175 | WFCJ, OPAL, VLA | Velocities, cloud structure/distribution |
F658N | 8 | 170 | OPAL | Cloud distribution/composition |
FQ727N | 8 | 150 | WFCJ, VLA | Cloud structure/distribution |
FQ750N | 6 | 180 | WFCJ, VLA | Cloud structure/distribution |
FQ889N | 15, 53 | 70, 130 | WFCJ, OPAL, VLA | Cloud/haze structure/distribution |
FQ906N | 15 | 170 | WFCJ | Cloud structure/distribution |
FQ924N | 12 | 170 | WFCJ | Cloud structure/distribution |
Download table as: ASCII Typeset image
2.1. Quad Filters
In WFC3, a range of methane-band filters are included as "quad" spectral elements, such that each one of them covers a quadrant of the overall WFC3/UVIS detector. Methane-band filters are centered on CH 4 gas absorption bands at 619, 727, and 889 nm. Within methane bands, light is only scattered back from high-altitude clouds, while images in continuum filters are unaffected by methane absorption and can, thus, detect deeper clouds. Additional narrowband filters were included in the WFC3 design with central wavelengths stepping along the edge of the strong methane band at 889 nm, specifically for the purpose of solar system and brown dwarf atmospheric studies (see Lupie et al. 2000 ). The availability of so many narrowband spectral elements is particularly beneficial for studies of aerosol vertical profiles (Table 1 ), but special care must be taken with target placement due to the quad nature of these filters.
Two constraints apply to target placement when using the quad filters: filter edge effects and guide star tracking. Near the inner boundaries of the quad filters lies a plus-shaped region (Figure 1 ) where data cannot be photometrically calibrated because the defocused, adjacent filters contribute light with blended spectral contributions. In normal use of these filters (i.e., positioning the target at the default reference point for quad subarrays, Section 6.4.5 of Dressel 2019 ), the target lies in the center of the area unaffected by the filter edges, and filter edge effects are minimized. We deviate slightly from this normal use in order to maximize guide star availability.
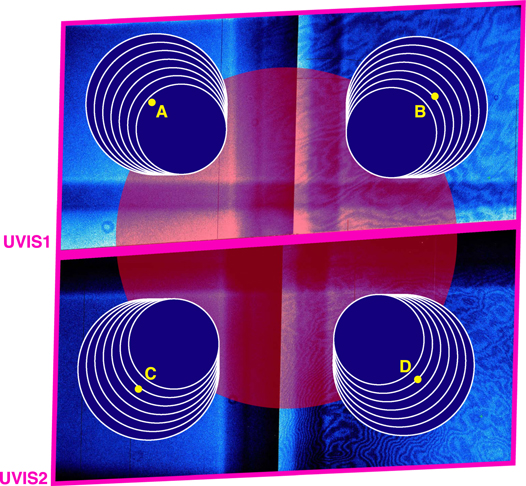
Figure 1. Targets in quad-filter exposures need to be carefully placed on the WFC3/UVIS detector in order to stay clear of filter edge effects (large central plus-shaped pattern in the blue background) but to keep slews inside a 120'' diameter region (large red central circle). If exceeded, the 120'' slew limit would trigger a new guide star acquisition (requiring 6 min overhead). The exact position adjustment depends on the size of the target (nested circles have diameters of 32''–50''). Reference points for each quadrant are labeled in yellow; Table 2 gives linear coefficients for offsets with respect to these reference points as a function of quadrant and target size. The figure is rectilinear in the sky coordinate frame, so the envelope of the UVIS detector forms a rhombus shape due to the tilt of the focal plane with respect to the optical axis.
Download figure:
Guide star availability becomes an issue when multiple quad filters are used, because any slew of 2' or more requires new guide stars to be acquired. The time needed to re-acquire guide stars reduces the amount of science time available in an orbit by about 8 minutes. Slewing between quad-filter reference points (yellow points in Figure 1 ) can easily exceed the 2' limit for using the same guide stars (red circle in Figure 1 ). Several programs use FQ727N, FQ750N, and FQ889N, which requires slewing the target to place it on quadrants D, B, and A, respectively (Table 2 ). In many cases, the target is also placed in quadrant C in order to use the subarrays defined there, thus introducing slews between all four quadrants. Balancing these two constraints (quadrant usage versus slew size) is improved by adjusting the target position with respect to the default reference positions for each quad aperture.
Table 2. Coefficients for Target Offsets a in WFC3/UVIS Quad-filter Exposures
Quadrant | POS TARG X | POS TARG Y | |||
---|---|---|---|---|---|
Relevant Filters | ('') | ('') | |||
A | FQ889N | −0.450 | +23.07 | +0.527 | −27.24 |
B | FQ750N | +0.537 | −34.46 | +0.465 | −26.79 |
C | FQ906N | −0.531 | +29.68 | −0.468 | +30.19 |
D | FQ727N, FQ924N | +0.453 | −28.25 | −0.532 | +32.22 |
2.2. Fringing
Fringing is a source of photometric error and large-scale pattern noise that affects narrowband filters at wavelengths >650 nm (Wong 2010 ). At long wavelengths, silicon becomes increasingly transparent, leading to constructive and destructive interference as incoming light experiences multiple internal reflections within the detector. The fringing amplitude has a strong dependence on the spectral energy distribution of the source convolved with the telescope throughput, and the pattern results from small variations in thickness across the detector. In Figure 1 , the fingerprint-like fringing pattern is stronger in quads B and D (750 and 727 nm) than in quads A and C (619 and 634 nm), due to silicon's transparency.
All long-wavelength narrowband WFC3 HLSPs described in Section 4 , as well as all long-wavelength narrowband OPAL maps described in Simon et al. ( 2015 ), have been corrected for fringing using preliminary "fringe flatfields" as described in Wong ( 2011 ). These assume a Jupiter spectral energy distribution based on the disk-averaged reflectance spectrum of Jupiter (Karkoschka 1998 ) convolved with the solar spectrum (Colina et al. 1996 ). The correction is not perfect, but some options for future improvements have been identified. The WFC3/UVIS detector thickness solution derived from monochromatic calibration images is inconsistent between the two main calibration image data sets (Wong 2011 ). This inconsistency may be ameliorated by applying a correction for the "flare" window ghost effect, as has now been done for WFC3/UVIS pipeline flatfields (Mack et al. 2016 ). Improvements to the optical-wavelength spectrum of Jupiter, particularly as it varies across the disk, may be expected from new hyperspectral observations (Dahl et al. 2018 ; Braude et al. 2020 ).
2.3. Geometric Distortion
Geometric distortion in raw WFC3 images is primarily caused by the tilt between the telescope beam and the detector plane. The coordinate transformation correcting for distortion is applied by the astrodrizzle task, which calls several reference files to determine the appropriate corrections. The astrodrizzle task is distributed within the AstroConda 12 package of analysis software currently supported by STScI. Polynomial and lookup-table corrections are provided by the IDCTAB and NPOLFILE reference files, respectively. Images are transformed from detector coordinates to sky coordinates in this astrodrizzle processing step.
Cosmic rays (and other transient nonideal pixel responses) affect many of the exposures. Frames with long exposure times are particularly susceptible to cosmic ray hits. Standard HST image processing is able to remove cosmic rays at the astrodrizzle step, by combining multiple images of fixed targets and by identifying cosmic rays as transient features. This approach cannot be used for image sequences of rotating bodies, especially when atmospheric change ensures that no two exposures are ever identical. Instead, we use the sharpness of the cosmic ray strikes themselves to clean them from single images, with the Laplacian edge-detection approach of van Dokkum ( 2001 ). Because the astrodrizzle distortion correction tends to blur the sharp edges of cosmic ray strikes, we must perform the single-image cosmic ray rejection procedure on UVIS data before correcting for distortion and transforming from detector coordinates to sky coordinates.
Distortion corrections differ slightly from filter to filter. Filter-dependent distortion solutions were used for the medium- and wide-band filters: filters ending with M or W in Table 1 . For the narrowband filters, the best available distortion solution was the one derived from the F606W filter (Kozhurina-Platais 2014 ). Images were processed with this distortion solution, and maps and other High Level Science Products (Section 4 ) were produced and uploaded to the MAST archive.
2.4. Shutter-induced Vibration
Planetary targets can be bright, and often require short exposure times (Table 1 ). For short WFC3/UVIS exposures, vibration from the shutter mechanism can degrade the image sharpness (Section 6.11.4 of Dressel 2019 ). For exposures shorter than 9 s, we specified the "A" side of the shutter blade, to minimize this effect. But in order to maximize the lifetime of WFC3/UVIS, operations are being changed as of mid-2019 to minimize mechanical movements caused when observers specify the A side of the shutter blade. Exposures longer than 5 s are deemed to be more strongly affected by focus changes due to breathing than by shutter-induced vibration.
2.5. Charge-transfer Efficiency
Charge-transfer efficiency (CTE) refers to the process of reading out the charge-coupled device (CCD) along parallel detector columns. As CCD detectors age (particularly in the harsh radiation environment of space), increasing numbers of photoelectrons become smeared out along the readout direction during detector readout, trailing behind the pixel where they were originally created. The HST observation planning tool (APT) generates warnings for observations that do not attempt to mitigate this issue (by flashing the detector with an internal LED lamp at the end of a science exposure). This post-flash operation evenly illuminates the whole detector, filling many charge traps and reducing inefficiency in the charge transfer.
We found that observations of bright extended targets do not require post-flash illumination, because the target effectively flashes the charge traps automatically. A substantial signal is carried in the extended wings of the point-spread function, so extended targets are surrounded by large halos. The halo is faint compared to the target itself, but it provides enough photons to fill charge traps in advance of any on-target pixels in the readout direction. Analysis of Uranus data (P. Fry 2015, private communication) showed that even the much fainter target suffered no astrometric error due to CTE effects.
Figure 2 shows the difference between uncorrected data (FLT files) and data with the pixel-based correction for CTE applied (FLC files; Baggett et al. 2015 ; Ryan et al. 2016 ). Comparing these two data products suggests that photometry on the planet's disk differs by 0.25% or less due to CTE effects (panel C). The position of the planetary limb is used for navigation and is found to be the half-power point between the on-planet brightness and surrounding space. The shifting of charge from the CTE correction acts to slightly move the half-power point in the readout direction. Panels (B) and (D) suggest this shift in the half-power point is in the range of 0.01 pixel for the sharp, illuminated limb, to 0.1 pixel for the darker terminator limb. This is smaller than our general navigational uncertainty, which has a precision of 0.25 pixel and an accuracy of ∼0.4 pixel. Because the pixel-based CTE correction is designed to improve photometry of sparse point sources rather than extended sources, we work exclusively with the uncorrected data (FLT files).
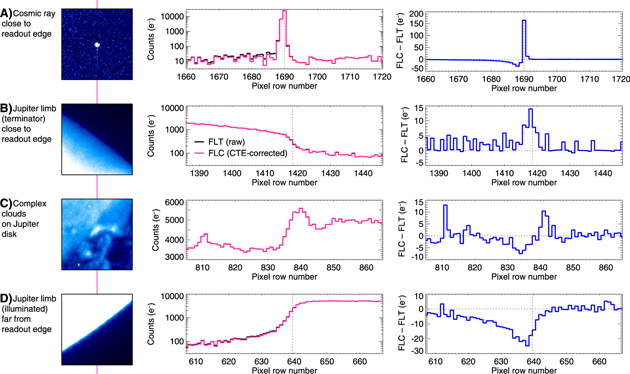
Figure 2. Charge-transfer efficiency has a minimal effect on photometry and astrometry for bright, extended sources like Jupiter. Each row shows a different detail of an HST image. The pink line behind the column of images indicates the column sampled in the plots at the right. Panel (A): A cosmic ray strike in a deep space part of the image leaves a trail of photoelectrons in the readout direction. The trail shows up as higher levels in the uncorrected FLT data (blue trace) compared to the corrected FLC data (pink trace). The difference plot in the right column shows that the correction takes counts out of the trail (negative values at rows <1690) and restores them to the core of the cosmic ray strike (positive spike). This is the desired result of the pixel-based CTE correction. Panel (B): Jupiter's limb (here, the terminator limb) needs to be precisely located for navigation and mapping of the data. The maximum correction of 14 e − (at row 1420, dashed vertical line) is about 5% of the signal, but does not noticeably affect the location of the limb. Panel (C): Complex cloud features have pixel-based CTE corrections less than 1% of the signal level (well within photometric uncertainties). Panel (D): Jupiter's illuminated limb is much sharper than the terminator limb in panel B. This section of the image is farthest from the readout edge of the detector and, thus, has the strongest CTE effects. Still, the correction is mainly concentrated in the deep space part of the image, and the limb location is identical in both corrected and uncorrected data.
2.6. Gyroscope Constraints
In order to manage gyroscope performance, additional observatory overheads were introduced in 2017–2018. Periodic gyro bias activities were required at regular intervals. Each gyro bias activity consists of a 20 minute procedure that must be done close to the same pointing as the target, but tracking at sidereal rates. The gyro bias activities reduce the number of science exposures per orbit that can be taken, so longitudinal coverage in some filters is less complete than other filters.
During Servicing Mission 4 in 2009, HST's complement of six gyroscopes was refreshed, with three standard flex lead gyros (G1, G2, and G5) and three enhanced flex lead gyros (G3, G4, and G6). Normal pointing and control is performed with three gyros operating simultaneously. At the beginning of 2018, HST was operating with G1, G2, and G4, but the performance of G2 was steadily declining. After failures of G1 (2018 April 21) and G2 (2018 October 5), HST is currently operating with its three remaining gyros: G3, G4, and G6 (Osten & Brown 2018 ; Osten 2019 ). All three are of the enhanced flex lead type that are expected to have greater lifetimes than the gyros that have failed to date. During the decline of G2 performance, and as stability issues with G3 were encountered, the number of consecutive HST orbits without a bias update evolved from eight orbits to four in 2017 June, and finally to two orbits in early 2018, affecting multi-orbit coverage of PJs 12 and 19, as well as OPAL 2018 and 2019 observations of Jupiter.
2.7. Photometric Calibration
The latest photometric calibration for WFC3/UVIS (Deustua et al. 2016 , 2017 ), called "UVIS 2.0," includes new flat fields and normalization procedures (Mack et al. 2016 ) compared to the earlier "UVIS 1.0" calibration pipeline in use prior to 2016. The WFC3 quad filters (i.e., FQ889N for the Jupiter observations discussed here) have not been updated, so we have included an estimated 3% reduction in calibrated fluxes in quad filters, based on average changes in photometric calibrations for other filters between the UVIS 1.0 and 2.0 calibration systems. Photometric uncertainties in the UVIS 2.0 calibration are estimated to be 1.2%–1.3% (Deustua et al. 2016 , 2017 ).
Calibrated HST data are images with data numbers corresponding to count rates in units of e − s −1 , and the FITS header keyword PHOTFLAM gives the inverse sensitivity factor to convert to spectral irrandiance units of erg cm −2 s −1 Å −1 . But for solar system science in the NUV–NIR range, reflectance in I / F units is commonly used. To convert the image data units of e − s −1 to I / F , we provide a FITS header keyword PHOTIF and its uncertainty, SIG_PHOT, such that
where Ω is the solid angle of a WFC3/UVIS pixel with default astrodrizzle parameters (0.03962 2 arcsec 2 ), and πF ⊙ is the solar spectral irradiance at Jupiter's orbital distance at the time of the exposure within the bandpass of the spectral element. To calculate πF ⊙ , we use the Colina et al. ( 1996 ) solar spectrum, the heliocentric distance from the JPL Horizons ephemeris system, 13 and spectral element throughput curves available directly for download from STScI. 14 The SIG_PHOT uncertainty in our I / F calibration is dominated by a systematic 5% uncertainty in the solar spectrum (Colina et al. 1996 ), but also includes a 1% error typical of photometric zero-point uncertainty (Deustua et al. 2017 ).
2.8. Temporal Coverage
Although most HST observations had timing linked to Juno perijoves, there are two exceptions. Observations in the Outer Planet Atmospheres Legacy (OPAL) program were taken near solar opposition, to maximize spatial resolution at Jupiter (Simon et al. 2015 ). OPAL 2017 observations happened very close to perijove 5.
A cluster of imaging observations in 2017 January were planned to coincide with a Juno perijove, under the original plan with 14 day Juno science orbits. When Juno was instead kept on a 53 day orbit (Bolton et al. 2017 ), the 2017 January observations at a number of observatories (including the VLA) were not reschedulable (de Pater et al. 2019b ). We include the 2017 January data from HST and Gemini in this report for two reasons: they have high intrinsic scientific value (in part due to the wide range of multiwavelength Jupiter observations planned during that time period), and they are still highly relevant to Juno because they document Jupiter's conditions as they evolve over the planned 5 yr Juno mission.
Table 3 lists the timing of both the HST and Gemini data reported here. The timing of Juno perijove passes is given in Table 4 . Some HST observations had to be offset by one or two Jupiter rotations due to limited guide star availability or due to accommodation of the Juno-related UV auroral imaging program (Grodent et al. 2018 ). Perijoves 5, 11, and 12 fell close to OPAL observations. Figure 3 gives a graphical summary of the temporal coverage of the data, compared to the Juno perijove sequence. On PJ 22, HST unfortunately missed the Juno longitude due to human error.
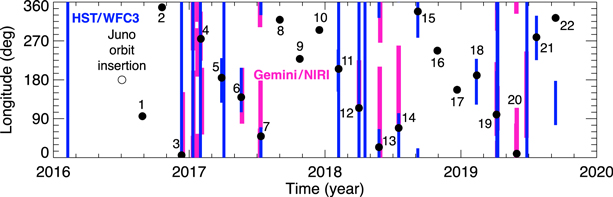
Table 3. Timing of HST/WFC3 and Gemini-N/NIRI M -band Imaging Observations
Juno PJ | Start (UTC) | Decimal Year | Span (hr) | Number of Frames | Instrument | Program ID |
---|---|---|---|---|---|---|
⋯ | 2016 Feb 09 09:35 | 2016.107 | 19.6 | 117 | WFC3 | GO-14334 |
3 | 2016 Dec 11 08:01 | 2016.943 | 25.6 | 172 | WFC3 | GO-14661 |
3 | 2016 Dec 14 14:21 | 2016.951 | 2 | 2 2 | NIRI | GN-2016B-FT-18 |
... | 2017 Jan 11 09:20 | 2017.029 | 14.9 | 124 | WFC3 | GO-14839 |
... | 2017 Jan 11 14:10 | 2017.029 | 2.4 | 2 2 | NIRI | GN-2016B-FT-18 |
... | 2017 Jan 12 13:03 | 2017.031 | 3.5 | 2 2 | NIRI | GN-2016B-FT-18 |
... | 2017 Jan 23 12:19 | 2017.062 | 4.2 | 2 2 | NIRI | GN-2016B-FT-29 |
4 | 2017 Feb 01 15:28 | 2017.086 | 21.4 | 154 | WFC3 | GO-14661 |
4 | 2017 Feb 01 15:40 | 2017.086 | 0.9 | 2 2 | NIRI | GN-2017A-Q-60 |
4 | 2017 Feb 05 14:12 | 2017.097 | 2 | 2 2 | NIRI | GN-2017A-Q-60 |
5 | 2017 Mar 27 07:03 | 2017.234 | 0.6 | 10 | WFC3 | GO-14661 |
5 | 2017 Apr 03 01:11 | 2017.253 | 21.2 | 105 | WFC3 | GO-14756 |
6 | 2017 May 19 14:38 | 2017.379 | 0.6 | 10 | WFC3 | GO-14661 |
6 | 2017 May 21 05:34 | 2017.384 | 1.4 | 3 2 | NIRI | GN-2017A-Q-60 |
7 | 2017 Jul 09 07:00 | 2017.518 | 1.3 | 2 2 | NIRI | GN-2017A-Q-60 |
7 | 2017 Jul 10 06:17 | 2017.521 | 0.9 | 2 2 | NIRI | GN-2017A-Q-60 |
7 | 2017 Jul 11 09:25 | 2017.524 | 0.6 | 14 | WFC3 | GO-14661 |
11 | 2018 Feb 06 17:20 | 2018.099 | 0.7 | 17 | WFC3 | GO-14661 |
11 | 2018 Feb 07 09:43 | 2018.102 | 8.2 | 44 | WFC3 | GO-14936 |
12 | 2018 Apr 01 08:38 | 2018.247 | 16.4 | 78 | WFC3 | GO-14661 |
12 | 2018 Apr 01 10:31 | 2018.247 | 0.8 | 3 2 | NIRI | GN-2018A-Q-202 |
... | 2018 Apr 17 01:15 | 2018.291 | 19.8 | 111 | WFC3 | GO-15262 |
13 | 2018 May 24 14:23 | 2018.392 | 0.6 | 10 | WFC3 | GO-14661 |
13 | 2018 May 26 07:38 | 2018.398 | 3.1 | 3 3 | NIRI | GN-2018A-Q-202 |
13 | 2018 May 27 10:17 | 2018.401 | 1.6 | 3 3 | NIRI | GN-2018A-Q-202 |
14 | 2018 Jul 16 06:00 | 2018.537 | 2.8 | 3 2 | NIRI | GN-2018A-Q-202 |
14 | 2018 Jul 16 13:47 | 2018.537 | 0.6 | 10 | WFC3 | GO-15159 |
15 | 2018 Sep 07 00:13 | 2018.682 | 0.7 | 6 | WFC3 | GO-14661 |
18 | 2019 Feb 12 15:59 | 2019.116 | 0.7 | 16 | WFC3 | GO-14661 |
19 | 2019 Apr 06 10:29 | 2019.261 | 3.8 | 18 | WFC3 | GO-15665 |
19 | 2019 Apr 06 12:23 | 2019.261 | 2.5 | 3 2 | NIRI | GN-2019A-Q-202 |
19 | 2019 Apr 07 10:19 | 2019.264 | 3.8 | 20 | WFC3 | GO-15665 |
19 | 2019 Apr 07 14:57 | 2019.264 | 0.7 | 3 2 | NIRI | GN-2019A-Q-202 |
19 | 2019 Apr 08 10:09 | 2019.266 | 3.8 | 20 | WFC3 | GO-15665 |
19 | 2019 Apr 08 14:21 | 2019.266 | 0.6 | 3 2 | NIRI | GN-2019A-Q-202 |
19 | 2019 Apr 09 08:23 | 2019.269 | 16.6 | 47 | WFC3 | GO-14661, 15159 |
19 | 2019 Apr 09 09:59 | 2019.269 | 3.8 | 19 | WFC3 | GO-15665 |
20 | 2019 May 28 12:38 | 2019.403 | 0.7 | 3 3 | NIRI | GN-2019A-Q-202 |
20 | 2019 May 29 09:30 | 2019.406 | 0.7 | 3 3 | NIRI | GN-2019A-Q-202 |
... | 2019 Jun 25 07:31 | 2019.480 | 3.3 | 3 3 | NIRI | GN-2019A-Q-304 |
... | 2019 Jun 26 08:14 | 2019.483 | 21.3 | 111 | WFC3 | GO-15502 |
21 | 2019 Jul 21 13:42 | 2019.551 | 0.6 | 10 | WFC3 | GO-14661 |
22 | 2019 Sep 12 08:04 | 2019.696 | 0.6 | 14 | WFC3 | GO-14661 |
Table 4. Juno Perijove Times and Geometric Parameters
Juno PJ | Time (UTC) | Decimal Year | Longitude (deg) | Solar Elongation (deg) | One-way Light Time (minutes) |
---|---|---|---|---|---|
JOI | 2016 Jul 05 02:47:32 | 2016.508 | 32.7 | 64.1 | 48.3 |
1 | 2016 Aug 27 12:50:44 | 2016.653 | 95.8 | 22.6 | 53.0 |
2 | 2016 Oct 19 18:10:54 | 2016.798 | 347.7 | 18.2 | 53.1 |
3 | 2016 Dec 11 17:03:41 | 2016.943 | 5.5 | 61.6 | 48.7 |
4 | 2017 Feb 02 12:57:09 | 2017.089 | 274.9 | 110.8 | 41.8 |
5 | 2017 Mar 27 08:51:52 | 2017.234 | 184.9 | 167.1 | 37.2 |
6 | 2017 May 19 06:00:47 | 2017.379 | 139.8 | 135.4 | 39.0 |
7 | 2017 Jul 11 01:54:42 | 2017.524 | 49.4 | 85.7 | 45.2 |
8 | 2017 Sep 01 21:48:50 | 2017.666 | 319.0 | 42.5 | 51.1 |
9 | 2017 Oct 24 17:42:31 | 2017.811 | 228.4 | 1.9 | 53.5 |
10 | 2017 Dec 16 17:56:59 | 2017.956 | 295.3 | 40.8 | 51.1 |
11 | 2018 Feb 07 13:51:30 | 2018.102 | 205.1 | 86.9 | 44.8 |
12 | 2018 Apr 01 09:45:43 | 2018.247 | 114.6 | 139.2 | 38.4 |
13 | 2018 May 24 05:39:50 | 2018.392 | 24.0 | 163.4 | 36.8 |
14 | 2018 Jul 16 05:17:39 | 2018.537 | 68.7 | 109.7 | 41.3 |
15 | 2018 Sep 07 01:11:57 | 2018.682 | 338.2 | 63.6 | 47.8 |
16 | 2018 Oct 29 21:06:17 | 2018.825 | 247.6 | 21.5 | 52.2 |
17 | 2018 Dec 21 17:00:27 | 2018.970 | 157.0 | 20.2 | 52.1 |
18 | 2019 Feb 12 17:34:16 | 2019.116 | 235.3 | 64.0 | 47.4 |
19 | 2019 Apr 06 12:14:00 | 2019.261 | 99.7 | 112.1 | 40.4 |
20 | 2019 May 29 08:08:14 | 2019.406 | 9.1 | 166.7 | 35.9 |
21 | 2019 Jul 21 04:02:44 | 2019.551 | 278.6 | 137.1 | 37.4 |
22 | 2019 Sep 12 03:40:47 | 2019.696 | 323.3 | 86.9 | 43.5 |
3. Gemini NIRI M -band Imaging Data
3.1. mosaic patterns.
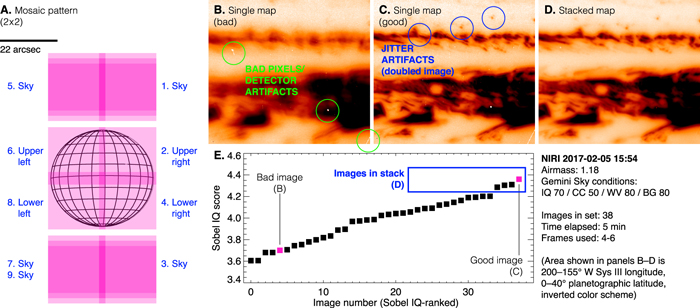
Interspersed with mosaic steps are sets of sky frames, needed to characterize the time-variable background brightness. Sky frames include 3'' offsets to eliminate any background sources that may be present, and a series of at least nine sky frames is needed to ensure that persistence (from the previous Jupiter frames) does not affect the sky exposures. In addition to creating background corrections, we used the sky frames to generate maps of dead and nonlinear pixels. In the final mosaics, these bad pixels were filled by dithering that was fortuitously introduced by telescope pointing jitter.
Mosaics were streamlined for 2018. Earlier observations (2016 and 2017) involved three sets of 38 exposures each at every mosaic pointing (with sky sets between each set). This ensured that variable sky conditions on timescales of 5–10 minutes did not adversely affect the data. But for later observations, we decided to prioritize efficiency by taking only a single set of 38 exposures at each mosaic pointing.
3.2. Lucky Imaging
Deciding which frames to use can be challenging, when hundreds of exposures are taken in a night. As a first step, we sort all 38 images taken at a single mosaic pointing, ranked by a custom Sobel Image Quality metric. The images ranked in the top 10% are examined to find the best single image, which serves as the key frame for the set.
Our Sobel IQ metric is based on the Sobel filter, an image transformation using a 3 × 3 pixel gradient operator to enhance edges in images (e.g., Danielsson & Seger 1990 ). We generate the Sobel IQ metric using the following steps:
- 1. Create a Sobel-filtered image from the key frame for a set of images.
- 2. Take a histogram of the pixel values in the filtered key frame image.
- 3. Determine the cutoff value within the filtered image. We empirically chose a value 10% larger than the half-width above the maximum in the histogram (approximately one sigma above the mean in the filtered image, if the distribution were Gaussian).
- 4. The number of pixels within the filtered image that are above the cutoff value is the Sobel IQ metric in our technique ( y -axis of Figure 4 ).
3.3. Temporal Coverage
Temporal coverage within the NIRI data set is given in Table 3 and Figure 3 . In some cases, offsets between Gemini and Juno timings by a couple of days were caused by unavailability of the NIRI instrument at Gemini North (it shares a port with NIFS), or due to difficulties observing the appropriate Juno longitude on Jupiter while the planet is at high enough elevation at night. Observations were attempted on 2018 February 9 near Juno's PJ11, but high winds rendered the data unusable (no sharp frames were obtained). The raw data are available in the Gemini archive, but these observations are not reported here due to their poor quality.
3.4. Additional High-resolution IR Imaging Programs
Observing programs are being conducted by other teams to image Jupiter during Juno spacecraft passes. The VISIR imager at ESO's Very Large Telescope (VLT), operated in burst mode to obtain lucky-imaging data also in the 5 μ m range, has obtained imaging data at several perijove times (Fletcher et al. 2018 ). This VLT data set includes longer-wavelength imaging, further discussed in Section 5.5 . NIRI is also being used to obtain near-IR images in reflected sunlight with the ALTAIR AO system, observing Jupiter at Juno-relevant times when Galilean satellites are available as natural guide stars (Giles et al. 2019 ). Data from the VLT and NIRI AO programs are not included as part of the archive collections described in Section 4 .
4. High-level Science Products in the WFCJ Archive Collection
A major motivation for this paper is to serve as a guide for HLSPs available from this program. The HLSPs are distributed across two nodes at STScI's MAST archive site. The Wide Field Coverage for Juno (WFCJ) program node contains HST and Gemini M -band HLSPs associated with Juno perijove passes, 15 including WFC3 and NIRI imaging data taken in direct support of VLA Jupiter observations. The OPAL program node contains HLSPs associated with annual Jupiter observations conducted near solar opposition for maximum spatial resolution. 16
The HLSP collections are dynamic and designed for growth, since new data for WFCJ will be collected over the Juno mission, and we expect that data for OPAL will be collected as long as HST's imaging capability remains functional. HLSPs available at these nodes are versioned, so older data are still available. The main interface serves the most recent version of all data products. New data products may be available in the future, but this paper describes the types of HLSPs currently available at these archive nodes. Figure 5 gives a graphical example of the content of the NAV HLSP type, and Table 5 summarizes the various types available:
- 1. NAV files are cleaned, I / F -calibrated, navigated individual image frames in sky coordinates. They are multi-extension FITS format binary files with extensions providing metadata, reflectance, latitude (planetographic), longitude, emission, and incident angle for each image pixel. The basic processing of HST data is performed with STScI packages available in the AstroConda distribution. Single images are cleaned as thoroughly as possible for cosmic rays using the LA-Cosmics routine based on Laplacian filtering (van Dokkum 2001 ), and corrected for fringing if necessary (Section 2.2 ). Geometric distortion is corrected as described in Section 2.3 . Navigation to sub-pixel accuracy is performed with the Simnav method (Lii et al. 2010 ), which aligns the real data with synthetic Jupiter images (including limb darkening and convolved with the WFC3/UVIS point-spread function obtained from TinyTim 17 ), based on geometric parameters from JPL Horizons.
- 2. REG files are individual regridded cylindrical projections of individual NAV files in longitude–latitude coordinates. REG files are multi-extension FITS format binary files with extensions providing metadata, emission, and incident angle. Latitude coordinates are in the planetographic system, but planetocentric maps can be provided upon request.
- 3. GLOBALMAP files are maps in longitude–latitude coordinates, combined from multiple cylindrically mapped exposures. Limb-darkening coefficients k are given in the README files associated with the data sets at the archive nodes, and k varies with filter (and sometimes with epoch). Values of k are chosen to maximize the aesthetic result in the GLOBALMAP output products. The limb-darkening functional form is a Minnaert function, as described in, e.g., Wong et al. ( 2018 ). The GLOBALMAP image sizes are selected to span 360° of west longitude and 180° of planetographic latitude. There are no emission/incident angle extensions in the GLOBALMAP files because viewing angle data have been corrected by the limb-darkening function. Theoretically, GLOBALMAP HLSPs could be generated by combining REG files, but practically, they have been generated for these programs by a separate process (Simon et al. 2015 ).
- 4. MOSAIC maps in longitude–latitude coordinates are similarly created from individual Gemini M -band maps. We do not host NAV or REG files from the Gemini data, because these are based on individual frames before stacking in the lucky-imaging approach (Section 3.2 ). We use a different name from GLOBALMAP for these products simply because it is rarely possible to create a full global map of Jupiter from a ground-based observatory. HST's 96 minute orbit provides an advantage in this respect over the 24 hr rotation period of the Earth.
- 5. POLAR files are polar-projected views of the north and south poles. Like NAV data, POLAR data are multi-extension FITS format binary files with extensions providing metadata, reflectance, latitude, longitude, emission, and incident angle for each image pixel. To ensure readability to the eye, individual frames and polar mosaics are all corrected for limb darkening, with limb-darkening coefficients encoded in the metadata. An additional backplane containing the original science data (without limb-darkening correction) is provided for single-frame polar projections. This backplane is not provided for polar mosaics, since those stack multiple frames at different original viewing geometries.
- 6. ZWP files are zonal wind profiles (ZWPs) derived by horizontal image correlation in data spanning two Jovian rotations. Thus, ZWPs are not available at every epoch. ZWP HLSPs are hosted in text (ASCII) and FITS format tables, with metadata and four columns of data. Column 1 is the latitude in planetographic coordinates. Column 2 is the eastward velocity in units of m s −1 . Column 3 is the uncertainty in the velocity. Column 4 is the number of tie points used to derive the uncertainty; a low number of tie points indicates a poorly determined uncertainty. The method for deriving ZWPs and estimating their uncertainties is described in Asay-Davis et al. ( 2011 ) and Tollefson et al. ( 2017 ). 18
- 7. MWRTRACKS are graphically shown on some preview images at the WFCJ archive node. These tracks indicate Juno's path over Jupiter's 1 bar surface during a specific perijove pass. Juno tracks are similarly shown in Figures 8 , 9 , and 11 in Section 5 , and in Figures 20–24 of Janssen et al. ( 2017 ). On passes optimized for MWR measurements, these tracks are composed of nadir footprints. On other passes, the tracks show minimum (but nonzero) emission angles observed by MWR during each spacecraft rotation. In many cases, the HST or Gemini images were acquired significantly before or after the Juno MWR measurements. In these cases, the footprint tracks have been advected by the ZWP to form somewhat twisted paths. For this purpose, we use the ZWP measured closest in time to the observations. The twisted paths pass over the same features in the map images that Juno measured (under the assumption that all motions are zonal).
- 8. COMPOSITES show multiple wavelengths of data mapped to the visual channels of the color image representations. Different filter mappings have been created to display optical color, cloud height, or ultraviolet reflectivity. Figures in Section 5 make use of color composites. Because a wide range of combinations can be created from the observations, composites are typically not archived as HLSPs. Instead, users may obtain data in separate filters and create composites on their own. One exception is RGB color composites (typically from F631N, F505N, and F395N) of GLOBALMAP data, which are available in TIF image format on the OPAL and WFCJ archive nodes. Composites may be created in sky coordinates to show Jupiter's full disk, or in longitude–latitude coordinates to show atmospheric maps. RGB color composites are simply created by loading one exposure per color channel, but more advanced methods (Rector et al. 2007 ) can be used to customize color schemes or display any number of images in separate wavelengths.
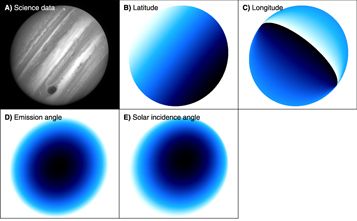
Figure 5. Several types of HLSPs available at the MAST archive include supporting data in backplanes (as FITS extensions). Shown here are backplanes of latitude, longitude, emission angle, and solar incidence angle data that are part of a NAV format HLSP. Details of each science product type are given in Section 4 and summarized in Table 5 .
Table 5. High-level Science Product Format Information
Science Product Type | Backplanes | Spatial Coordinates | File Format | Source | Archive Node |
---|---|---|---|---|---|
Latitude | |||||
NAV | Longitude | Sky | FITS | WFC3 | WFCJ |
Emission angle | |||||
Incidence angle | |||||
REG | Emission angle | Latitude/longitude | FITS | WFC3 | WFCJ |
Incidence angle | |||||
GLOBALMAP | None | Latitude/longitude | FITS, TIFF, PNG, JPG | WFC3 | OPAL, WFCJ |
MOSAIC | Emission angle | Latitude/longitude | FITS, JPG PDF | NIRI | WFCJ |
Incidence angle | |||||
Latitude | |||||
Longitude | |||||
POLAR | Emission angle | Polar-projected latitude/longitude | FITS, JPG | WFC3 | WFCJ |
Incidence angle science image | |||||
ZWP | None | Latitude | ACII, FITS | WFC3 | WFCJ |
MWRTRACKS | None | Latitude/longitude | PNG, JPG | Juno | None |
Although this paper provides an overview of the data set and a thorough description of the data reduction processes, early releases of the data have already been published in focused scientific studies. In this section, we describe some of the early science results and provide updates to ongoing research in the studies of zonal winds, atmospheric waves, convective storms, the GRS, cyclonic vortices, and polar phenomena.
5.1. Zonal Winds
ZWPs derived from programs listed in Table 3 have been recently published. Tollefson et al. ( 2017 ) analyzed the temporal variability of Jupiter's ZWP, extending the results of Simon-Miller & Gierasch ( 2010 ) and Asay-Davis et al. ( 2011 ). Notably, Tollefson et al. ( 2017 ) demonstrated mean uncertainties of ∼6 m s −1 in the zonal wind speed using WFC3 data, about a factor of two better than was possible with the previous-generation WFPC2 camera on HST. Johnson et al. ( 2018 ) quantified spatial variation in zonal flow, finding significant changes in jet speeds and latitudes at different locations around the planet, and Hueso et al. ( 2017 ) showed consistency between ZWPs derived from 2016 WFC3 and ground-based imaging (albeit with a factor of two larger standard deviation in the ground-based profile).
Simon-Miller et al. ( 2007 ) and Simon-Miller & Gierasch ( 2010 ) found hints of periodic variation in Jupiter's ZWP, depending on the data sets included in the analysis. They used Lomb–Scargle periodograms to search for significant periodic signals at specific latitudes. One particular issue was limited coverage of short-timescale variability. A significant equatorial variation with a period near 12 yr was seen in a 14 yr HST/WFPC2 data set that included a 2007 March ZWP, but it was not seen in an identical data set that included 2007 February instead of 2007 March (Simon-Miller & Gierasch 2010 ). Tollefson et al. ( 2017 ) found a similar significant equatorial periodicity (at 13.8 yr instead), using a 22 yr data set that combined ZWPs derived from both WFPC2 and WFC3 data. Given the influence of data sets with short time separations on the periodogram results, we used several new ZWPs derived from WFC3 data to augment the Tollefson et al. ( 2017 ) data set, reaching a total duration of 25 yr (excluding Voyager) and containing more short time separations within the 2017–2019 period. Figure 6 shows the time series and resulting periodograms, using all available data (top) or a subset of data omitting any ZWPs within 5 months of another ZWP closer to opposition. The particular ZWPs used in Tollefson et al. ( 2017 ), and in each row of Figure 6 , are listed in Table 6 . Periodograms corresponding to additional subsets listed in Table 6 are available in the Appendix . The periodograms are based on ZWPs like those in Figure 7 (A), smoothed to one-degree latitudinal resolution.
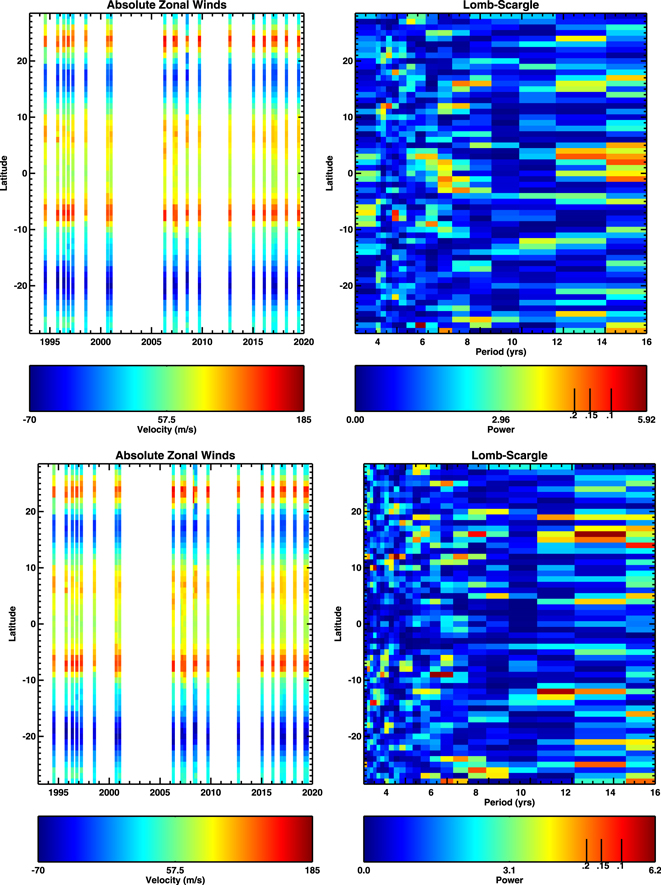
Figure 6. Different groups of zonal wind profiles data sets show consistent signs of periodic variability (see Section 5.1 ). Top panels: All ZWPs from Voyager in 1979 to HST in 2019 are used. Bottom panels: ZWPs are omitted when they are separated by <5 months from another profile and when they are derived from lower-resolution data than the other profile (column labeled "Subset" in Table 6 ). Left column panels: Zonal winds from spacecraft imaging obtained with Voyager, Cassini, and HST, with wind speeds as a function of time and latitude shown as color values. Right column panels: Lomb–Scargle periodograms based on the wind profiles, with power as a function of period and latitude shown as color values. False alarm probabilities of 20%, 15%, and 10% are shown as vertical ticks on the color bar. Individual periodogram pixels are centered on their periods.
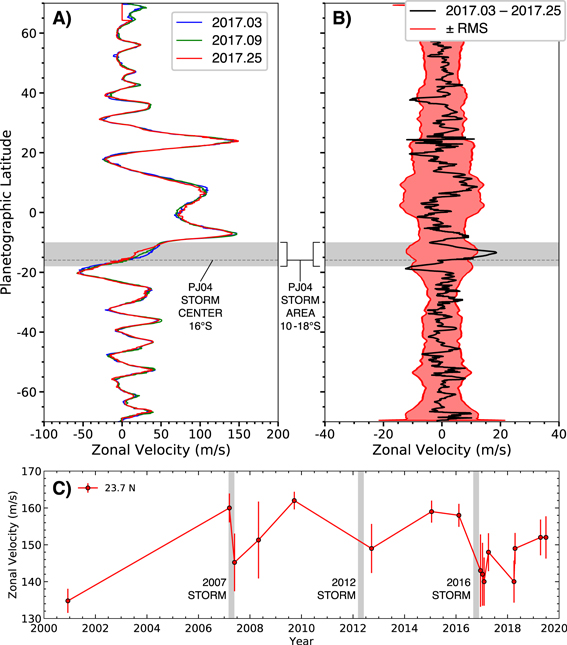
Table 6. Zonal Wind Profiles Included in Tollefson et al. ( 2017 ), Figure 6 ("All" and "Subset" Columns), and Figures 14 – 20 in the Appendix (Remaining Columns)
Date | Tollefson et al. ( ) | All | Subset | Subset 2018 | HST Only | WPC2 + WFC3 | WFC3 only | HST Only (Filtered) | WFPC2 + WFC3 (Filtered) |
---|---|---|---|---|---|---|---|---|---|
1979.42 | X | X | X | X | |||||
1994.55 | X | X | X | X | X | X | X | X | |
1995.76 | X | X | X | X | X | X | X | X | |
1996.37 | X | X | X | X | X | X | X | X | |
1996.81 | X | X | X | X | X | X | X | X | |
1997.26 | X | X | X | X | X | X | X | X | |
1998.54 | X | X | X | X | X | X | X | X | |
2000.68 | X | X | X | X | X | X | X | X | |
2001.02 | X | X | X | X | |||||
2006.31 | X | X | X | X | X | X | |||
2007.16 | X | X | X | ||||||
2007.23 | X | X | X | X | X | X | X | X | |
2007.43 | X | X | X | X | X | X | X | X | |
2008.38 | X | X | X | ||||||
2008.52 | X | X | X | X | X | X | X | X | |
2009.72 | X | X | X | X | X | X | X | X | X |
2012.72 | X | X | X | X | X | X | X | X | X |
2015.05 | X | X | X | X | X | X | X | X | X |
2016.11 | X | X | X | X | X | X | X | X | X |
2016.95 | X | X | X | X | X | X | X | X | X |
2017.03 | X | X | X | X | |||||
2017.09 | X | X | X | X | |||||
2017.25 | X | X | X | X | X | X | X | X | |
2018.25 | X | X | X | X | |||||
2018.29 | X | X | X | X | X | X | X | X | |
2019.27 | X | X | X | X | X | X | X | ||
2019.49 | X | X | X | X |
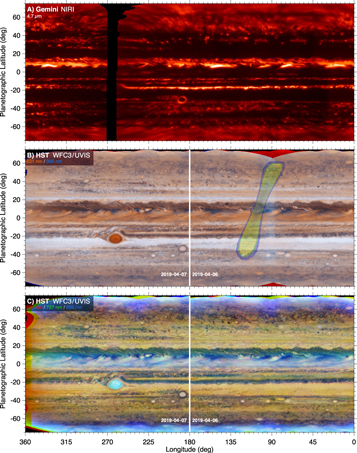
Figure 8. At the time of Juno's 2019 cross-track orbit (see Figure 21 of Janssen et al. 2017 ), Gemini (panel A), the VLA (not shown), and HST (panels B and C) mapped Jupiter one hemisphere at a time over two nights. Juno MWR footprints are displayed in panel (B). Near closest approach the footprints show that MWR mapped the western half of a 5 μ m hot spot, and most likely caught one of the ammonia-rich "plumes" associated with the equatorially trapped Rossby wave (de Pater et al. 2016 ).
Nonperiodic changes are also evident in Jupiter's zonal winds. Figures 7 (A), (B) shows an example of ZWP changes in 2017, following a system of storms known as a South Equatorial Belt (SEB) Outbreak. A kink in the ZWP is commonly seen in the 10°–15°S area of the SEB. A series of 2017 ZWP measurements shows that following the SEB Outbreak, the kink narrowed and shifted southwards. The three ZWPs changed monotonically over a period of almost 3 months, although only the change from 2017.03 to 2017.26 was significant beyond the formal uncertainties in the ZWP. The nature of this kink, which is unique except for a possibly similar feature in the cyclonic region between 23° and 30°N, bears further investigation in the future. The changes in the ZWP could be related to vertical wind shear revealed by changing cloud deck levels, variability across longitudinal sectors, or true changes in the overall wind speeds.
Wind speeds near 24°N are also affected by major convective outbreaks (Figure 7 (C)). As reported in previous works, the peak jet speed increases to its maximum before one of these storm events, then drops dramatically after North Temperate Belt Outbreaks occur (Sánchez-Lavega et al. 2008 , 2017 ; Hueso et al. 2017 ; Tollefson et al. 2017 ).
A practical application of ZWPs is to compare observations taken at slightly different times. We use the zonal winds to "advect" observation footprints from one observation to match nonsimultaneous imaging coverage. Figures 9 and 11 give examples of this.
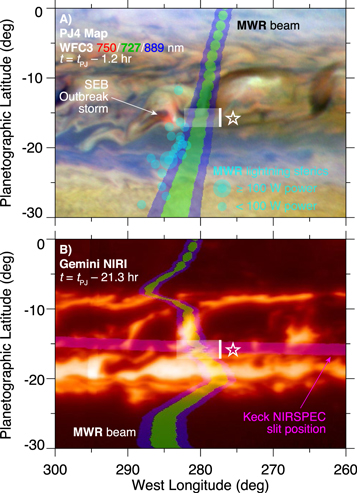
Figure 9. Juno and supporting context observations combine to reveal the most comprehensive picture yet of the convective process on Jupiter, in an example from PJ 4. Panel (A): HST multispectral imaging shows the presence of deep water clouds (in red) near the Juno MWR track (green/blue stripe). At the location marked by a white star, Juno MWR retrievals of NH 3 mixing ratio show strong ammonia depletion in the inter-storm region (Bellotti & Steffes 2017 ), a marker of downwelling flow consistent with dynamical models (Li & Ingersoll 2015 ). Lightning flashes detected by Juno MWR (Brown et al. 2018 ) are shown as cyan circles, each plotted at the MWR boresight pointing at the time of a lightning flash (Brown et al. 2018 ). Lightning is consistent with deep (water cloud level) convection in this storm. Panel (B): Gemini NIRI imaging shows regions of low cloud opacity (bright) between storm cores. The pink stripe shows Keck NIRSPEC slit position for high-resolution 5 μ m spectroscopy (Bjoraker et al. 2018a , 2018b ). The footprint shows the potential of the 5 μ m spectral data to constrain the NH 3 depletion independently from Juno MWR but modeling is not yet complete.
5.2. Atmospheric Waves
Mesoscale waves with wavelengths of ∼1° (1200 km) in Jupiter's North Equatorial Belt (NEB) were seen in Voyager images and rediscovered in 2015 HST images (Simon et al. 2015 ). The waves were absent in intervening years (except for a possible sighting in 2012). Since 2015, these mesoscale waves have been seen in many other data sets, even in imaging by amateur astronomers. A comprehensive study of the conditions over which these features were present from 2015 to 2018, using visible wavelength data from both HST and ground-based facilities, found that the waves were most commonly present near interacting vortices in the NEB (Simon et al. 2018a ). Specifically, they seemed to be forming to the west of prominent cyclones, and these cyclones form in former locations of prominent "bulges" of the NEB associated with an expansion episode (Fletcher et al. 2017 ). The Gemini 5 μ m data, along with extensive 5 μ m imaging from the VLT and Juno's JIRAM instrument, demonstrated that these waves modulated cloud opacity in the 0.5–2 bar range (Adriani et al. 2018a ; Fletcher et al. 2018 ). The wave properties are consistent with inertio-gravity waves.
Rossby waves are much larger, planetary-scale systems that are confined to propagate in the east–west direction by the Coriolis force. The best-known example of Rossby waves on Jupiter is the system of 5 μ m hot spots in the southern part of the NEB, near 7°N (e.g., Ortiz et al. 1998 ). Both HST and Gemini components of this data set were used by Marcus et al. ( 2019 ) to investigate the properties of 5 μ m hot spots, and characterize the velocities in and around them. The 5 μ m hot-spot Rossby wave system is the deepest known wave in Jupiter's atmosphere, modulating deep NH 3 concentrations as shown by microwave and millimeter wave maps from the VLA and ALMA (de Pater et al. 2016 , 2019a , 2019b ). This deep Rossby wave extends all the way up to the upper troposphere, as indicated by variations in the ammonia concentrations there retrieved from IRTF/TEXES data (Fletcher et al. 2016 ). Our UV/visible/IR imaging data will be valuable for comparison with Juno MWR data acquired during PJ19, the cross-track mapping perijove. Juno's scans over the NEB covered one of the 5 μ m hot spots, and most likely its adjacent "NH 3 -plume" (de Pater et al. 2016 ). Figure 8 shows that not all 5 μ m hot spots are created equal; the hot spot scanned by Juno on PJ19 was not one of the infrared-brightest on that date. The relative brightness of these features is known to vary spatially as well as temporally (Ortiz et al. 1998 ; Orton et al. 1998 ).
In contrast to this deeply seated wave system, a high-altitude Rossby wave system slightly to the north near 13°N is rendered visible by its modulation of haze altitude levels (Giles et al. 2019 ). HST maps from 2017 April (Table 3 ) provided context for the upper-tropospheric wave system described by Giles et al. ( 2019 ), based on IRTF near-infrared imaging.
5.3. Convective Storms
Very large convective outbreaks on Jupiter are relatively rare, but the prevalence of lightning over the planet suggests that moist convection takes place much more frequently in smaller storms. Spacecraft imagers have detected lightning distributed all over the planet, but more concentrated in regions of cyclonic zonal wind shear (Little et al. 1999 ). Lightning is thought to be much more likely in the presence of mixed condensate phases (Levin et al. 1983 ), and water is the only liquid condensate thought to form in Jupiter's troposphere. Analysis of lightning flash geometry is consistent with deep flashes that occur at levels corresponding to the water cloud layer (Dyudina et al. 2002 ; Wong et al. 2008 ). Unlike Saturn, where radio emissions show that lightning is not a continuously occurring phenomenon (Dyudina et al. 2007 ; Sayanagi et al. 2013 ), Jupiter's sferics and whistlers agree with prior imaging results, in that lightning has been detected during every Juno pass, and broadly distributed over the planet (Brown et al. 2018 ; Imai et al. 2018 ; Kolmašová et al. 2018 ).
During PJ 4, Juno passed close to a somewhat smaller (but still enormous) convective storm in the SEB. This storm, a plume within a series of convective pulses that are collectively known as an SEB Outbreak, was imaged by HST and Gemini, providing context for Juno MWR measurements in its vicinity (Figure 9 ). In particular, a strong local depletion of ammonia in the 1–2 bar altitude range was detected by Juno MWR (Bellotti & Steffes 2017 ), at the location indicated by a white star in Figure 9 . HST and Gemini high-resolution imaging contribute to interpretation of the results by showing that the depleted region corresponds to a dark, cloud-free region in between storm pulses. Atacama Large Millimeter/submillimeter Array (ALMA) observations of the SEB Outbreak system three months prior show that the ammonia depletion is not only present between storm plumes but in fact encircles active storm plumes (de Pater et al. 2019a ). The low NH 3 concentration in this inter-storm region is consistent with the numerical model of Li & Ingersoll ( 2015 ), which also produced volatile-depleted downwelling regions in the periphery of convective storms. Radio signals detected by MWR reveal a large number of lightning flashes in the vicinity of the storm (cyan circles in Figure 9 (A)). Precise location of the lightning flashes is challenged by the 20° beam size of the MWR at the lowest frequency (Brown et al. 2018 ), but many of the flashes may have been associated with a deep water cloud that is revealed by HST data.
5.4. The Great Red Spot
The GRS has been shrinking for over a century, and its color has also intensified over the past decade (Asay-Davis et al. 2009 ; Shetty & Marcus 2010 ; Simon et al. 2014 , 2018b ). The increased frequency of HST Jupiter observations during the Juno mission means that additional data are now available to characterize much shorter-term changes in the GRS, such as a 90 day oscillation in its drift rate (Reese 1971 ; Trigo-Rodriguez et al. 2000 ).
New, unexplained features in the GRS are revealed by comparing simultaneous 5 μ m and visible imaging. In Figure 10 , holes in the clouds show up as bright features at 5 μ m and dark features at 631 nm. High-resolution 5 μ m imaging is rarely conducted, so it is not clear how rare these features are, and whether they signal a significant change in the GRS cloud layers. However, high-resolution 5 μ m images obtained with adaptive optics at the Keck Observatory did not detect these interior cloud gaps in 2006 or 2008 (de Pater et al. 2010 ). The Keck imaging data led to a conclusion that large anticyclones (with radius > L R ) like the GRS and Oval BA lack broad, continuous 5 μ m bright rings, while small anticyclones are completely encircled by 5 μ m bright rings of low cloud opacity. The Rossby deformation radius, L R , is the characteristic length scale for geostrophic balance between buoyancy and Coriolis forces (Pedlosky 1987 ). The 2006 images did however show thin, incomplete 5 μ m arcs to the south of the GRS and Oval BA in 2006 but not 2008. The situation is different in the 2018 map of Figure 10 , with a much more extensive southern arc composed of several concentric thin arcs and bright spots, possibly related to low-opacity regions in the northern part of the vortex that lie at similar distances from the vortex center. The main evidence for the de Pater et al. ( 2010 , 2011 ) hypothesis that anticyclone circulation is fundamentally different in vortices larger or smaller than L R , was a difference in the 5 μ m ring morphology for large and small anticyclones. In light of the evolving partial rings around the GRS and the full ring around Oval BA in Figure 8 , this hypothesis may be challenged.
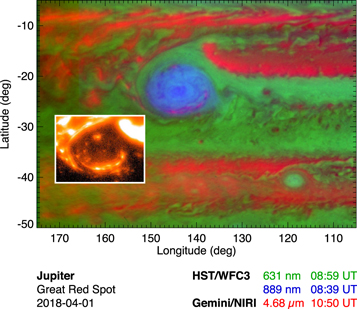
Figure 10. Nearly simultaneous Gemini and HST views of the GRS reveal the nature of dark lanes. These features are dark at visible wavelengths (green channel in composite map), and had previously been explained as either dark cloud features or areas of reduced cloud opacity (Sánchez-Lavega et al. 2018 ). Bright spots in the 5 μ m map (shown by itself in the inset) are only consistent with the second explanation. The enhanced GRS haze (blue channel) is uncorrelated with these areas of reduced cloud opacity.
Sánchez-Lavega et al. ( 2018 ) described similar "dark filaments" in the JunoCam images of the GRS taken in 2017 July at PJ07. They likened the features to "dark lanes" seen in Voyager, Galileo, and Cassini imaging (e.g., Simon-Miller et al. 2001 ), but their radiative-transfer analysis could not distinguish between two explanations: areas of reduced cloud opacity or areas with darker cloud material. The simultaneous visible and 5 μ m imaging shown in Figure 10 clearly shows that at least during PJ 12, the dark filaments or dark lanes result from reduced cloud opacity.
5.5. Cyclonic Vortices
Within the set of Juno MWR data up to PJ 8, the largest cluster of lightning flashes was detected during PJ 6, near 45°–50°N. Figure 11 compares the approximate location of lightning flashes with the cloud features visible to HST one Jupiter rotation later. Locations plotted are the boresight pointing positions at the time each lightning sferic was recorded by Juno's MWR channel 1, but there is some uncertainty within the antenna beam. The 20° MWR channel 1 beam size (as projected on Jupiter) can be estimated by the width of the blue stripe in Figure 11 ; this shows the FWHM at the minimum emission angle sampled during each spacecraft rotation. The longitudinal width of the beam increases substantially at higher emission angles, due to perspective from the spacecraft's vantage point. Sizes of markers of lightning sferics in Figure 11 distinguish between flashes with energies above and below 100 W minimum effective isotropic radiating power. The energy estimates are lower limits because if the actual lightning flashes were located away from the boresight location, then their actual power would have been greater. Lightning locations and power estimates are taken from supplemental materials published with Brown et al. ( 2018 ).
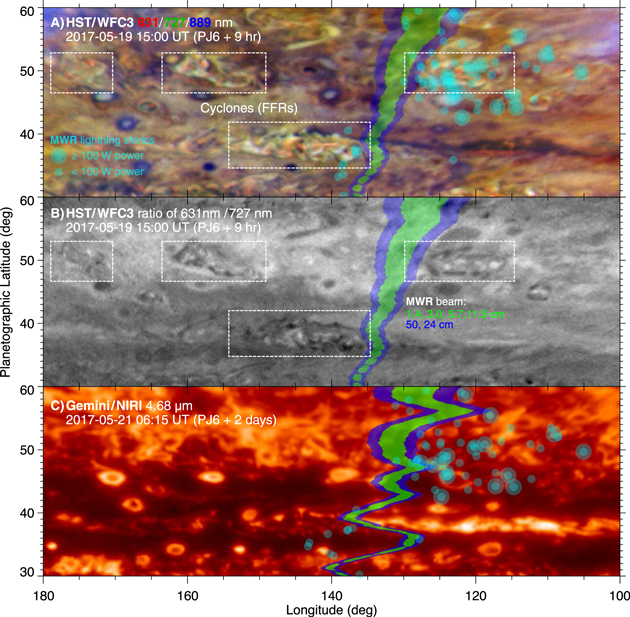
Figure 11. Cyclones (some enclosed in dashed white boxes) frequently have deep clouds ( P ≥ 4 bar), probably composed of water liquid and/or ice. During PJ 6, Juno passed close to several cyclones. Panel (A): Cloud heights are represented as color in a composite of HST data in a deep-sensing continuum filter (631 nm), and weak and strong methane bands (727 and 889 nm) that sense cloud opacity at P < 4 bar and P < 0.6 bar, respectively (see Section 5.5 for discussion of uncertainties in cloud opacity pressure levels). Panel (B): The ratio of HST 631/727 nm reflectivity is displayed as a high image brightness for deep clouds, and a low brightness for high-altitude clouds. Panel (C): Gemini 4.8 μ m radiance is inversely related to cloud opacity in the 1–5 bar range. The Juno minimum-emission-angle footprint track is shown in blue and green. The Juno footprints are advected by zonal winds to account for the time delay between the spacecraft pass and the HST/Gemini imaging. The blue contour shows the variation of MWR Channel 1 beam size (half-power radius) with latitude; this is the channel most sensitive to lightning sferics. Cyan dots indicate the Channel 1 boresight position at the time of each lightning flash; the offset between the actual position of lightning flashes and the boresight pointing affects the derived effective isotropic radiating power, but this offset cannot be precisely determined. Longitudes of boresight positions have also been advected to compensate for the delay between the Juno pass and the HST/Gemini imaging. In panels (A) and (B), brightness gradients as a function of latitude have been removed (to emphasize longitudinally discrete features) by dividing the data in each latitude bin by the mean at that latitude.
A large number of the sferics detected in PJ 6 are associated with cyclonic vortices. Cyclonic circulation is an assumption based on the appearance of the cloud features. Only a single HST orbit was used to observe Jupiter at PJ 6, so actual velocities could not be measured to demonstrate cyclonic (counterclockwise) rotation. However, similar features (known as folded filamentary regions, or FFRs) with these types of fine-scale disorganized features, confined to a circular or elongated region, previously have been observed to rotate cyclonically. The connection between lightning, moist convection, and cyclones was discussed in Fletcher et al. ( 2017 ), following ideas that a statically stable convective inhibition layer (Guillot 1995 ; Sugiyama et al. 2014 ; Leconte et al. 2017 ) is perturbed/weakened in low-pressure cyclones and regions with cyclonic zonal wind shear (Thomson & McIntyre 2016 ).
In the color scheme of Figure 11 (A), the deepest clouds (potentially water clouds) appear red. Green and blue channels in the composite are taken in weak and strong methane bands, respectively, so that red clouds are deep, yellow clouds have significant opacity at P < 4 bar, and blue regions have strong upper-tropospheric haze opacity. White clouds in this scheme are thick clouds that also reach exceptionally high into the upper troposphere. In Figure 11 (B), we map the continuum-to-weak CH 4 -band reflectance ratio ( I/F 631 nm / I/F 727 nm ), following the approaches of Banfield et al. ( 1998 ) and West et al. ( 2004 ). This ratio has high values for deep ( P > 4 bar) clouds and low values for higher-altitude clouds. Within the cyclonic FFRs (dashed boxes), compact deep clouds (red in Figure 11 (A) and bright in Figure 11 (B)) appear near the centers of the features, while thick clouds that reach high altitudes (white in Figure 11 (A) and dark in Figure 11 (B)) are more typically found near the outer edges. It is not obvious whether the lightning flashes reported in Brown et al. ( 2018 ) are associated with the compact deep clouds or the thick high-altitude clouds.
The presence of water clouds in these cyclonic vortices, particularly in the cyclones with strong lightning activity, is significant because lightning strongly favors mixed phase (liquid and solid) cloud particles (Levin et al. 1983 ). It is important to note that the pressure level of clouds that appear in continuum wavelengths (631 nm or 750 nm), but that do not appear the weak methane band (727 nm), can only be constrained by detailed radiative-transfer modeling beyond the scope of this paper. The determination that bright features in Figure 11 (B) are located at P > 4 bar is based on the analysis of Li et al. ( 2006 ) that the 727 nm filter of Cassini/ISS reached the τ = 1 level at 4 bar. However, this value is affected by viewing geometry, differences in filter bandpass between Cassini/ISS and HST/UVIS, and the presence of overlying haze and thin cloud layers. Detailed radiative-transfer analyses have been done with 727 nm and continuum maps using Galileo/SSI data, finding clouds at P > 4 bar in the vicinity of convective storms (Banfield et al. 1998 ; West et al. 2004 ).
Figure 12 compares three types of Jovian cyclones, in a southern-hemisphere view. FFRs near 47°S, similar to those with strong lightning activity in PJ 6, again are seen to have deep water clouds. Although we cannot directly identify the composition of these clouds based on multi-filter imaging, their location at P > 4 bar suggests that they are too deep to be composed of NH 4 SH or NH 3 ices (Weidenschilling & Lewis 1973 ; Atreya & Romani 1985 , Wong et al. 2015 ). Similarly, another type of cyclone labeled "barge" has a central water cloud. A long-lived vortex labeled "Spectre," on the other hand, does not have detectable water clouds. But unlike the FFR and barge cyclones, the Spectre does not have regions clear of overlying cloud opacity in the P < 4 bar range, so any water clouds that may be present would not be detectable. Thermally, all three types of cyclones are associated with warm anomalies near the 0.5 bar level, as shown by the mid-IR map at 10.8 μ m. This wavelength is sensitive a combination of ammonia gas, aerosol opacity, and temperature, all consistent with downwelling flow. Haze distributions are valuable probes of the upper levels of these features. The strong methane-band (FQ889N) map shows that these cyclones are locally depleted in haze particles, but the UV images do not show a similar depletion. This difference is most likely the result of differences in the haze particle size distribution, with the larger haze particles detected near 890 nm more strongly affected by the cyclonic vortex than the small particles detected in the UV.
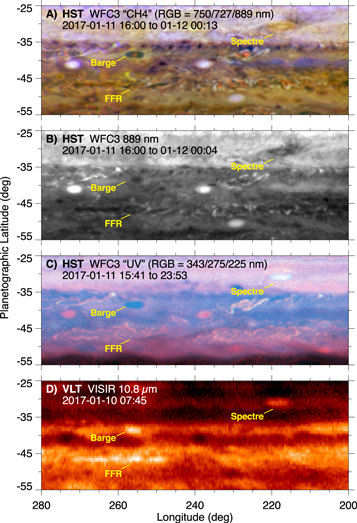
Figure 12. Types of cyclones on Jupiter have diverse appearances at wavelengths from the UV to the mid-IR. Panel (A): A composite of continuum, weak, and strong methane-band maps reveals differences in cloud heights. The deepest clouds appear only in the continuum channel (red), and must be located at P > 4 bar. The only clouds expected to condense this deep are composed of H 2 O. The folded filamentary region (FFR) cyclone and the barge both have visible water clouds, but thick high-level clouds prevent any water clouds from being directly observed in the Spectre. Panel (B): The strong methane band is shown alone, to emphasize that all three types of cyclones have reduced upper-tropospheric haze reflectivity, relative to their surroundings. Panel (C): In the UV, there is no significant depletion of upper-tropospheric haze, indicating that the smallest particles (not sensed at 889 nm in panel B) are not destroyed or redistributed by cyclone dynamics. Panel (D): Mid-IR maps—sensitive to a combination of tropospheric temperature, ammonia, and aerosols near the 500 mbar level—show similar anomalies associated with all three cyclone types.
5.6. Polar Phenomena
Full global coverage was achieved at many of the observational epochs, enabling the polar regions to be mapped. Figure 13 gives an example of the north polar haze structure in 2017 January. "Polar" hazes extend as far south as 35°N, and as far north as 50°S. The color-composite map in Figure 13 (A) demonstrates significant changes in haze properties every ∼10° of latitude in the wavelengths shown, between 40°N and 80°N. Detailed radiative-transfer modeling beyond the scope of this paper is needed to understand what causes these meridional changes in haze properties, but multiple effects are probably at work. Each filter has different vertical sensitivity, so vertical layering of polar hazes may explain some of the meridional variation. Hazes are bright in the near-IR CH 4 band, but absorbing at UV wavelengths, so some of the reflectivity variation is linked to composition. Particle size effects are also significant across such a wide range of wavelengths. Much of the polar stratospheric haze is thought to be generated by methane photolysis, driven by solar UV (West et al. 1986 ). Differences in stratospheric composition at high latitudes have been measured by Cassini CIRS (Nixon et al. 2007 ). But auroral chemistry may also contribute to the haze cap north of 70°N, within which a UV-dark oval has been reported at some epochs (Porco et al. 2003 ; West et al. 2004 ).
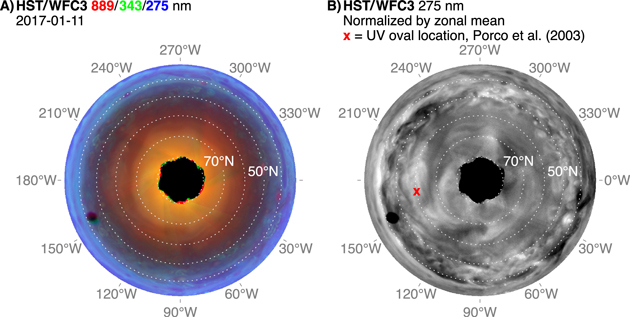
Figure 13. A north polar map shows the complex structure of polar haze "caps," in a global mosaic composed of observations from 2017 January 11. Limb darkening has been removed from each individual frame. Panel (A): Color map combines CH 4 -band data (889 nm) in the red channel, with UV data (343 and 275 nm) in the green and blue channels. At 275 nm, the smallest haze particles, with strong UV absorption, increase in opacity near 40°N and again near 50°N. Similar boundaries are apparent at 343 nm, but the 275 nm/343 nm color ratio is different in these two concentric haze layers. The northernmost polar haze cap, with a boundary near 70°N, is characterized by strong reflectivity at 889 nm and 343 nm, but is hardly discernible at 275 nm or 225 nm (not shown). Each concentric polar haze cap has wave-like structures around its boundary, and the innermost cap near 70°N is suggestive of a somewhat irregular decahedral or hendecahedral pattern. Panel (B): The 275 nm mosaic has been normalized by the zonal mean at each latitude, revealing longitudinal variation in reflectivity. The dark UV oval described in Porco et al. ( 2003 ) and West et al. ( 2004 ) is absent at this epoch. The shadow of Ganymede can be seen in the mosaics near 155°W and 45°N.
6. Conclusions
Our imaging context from HST and Gemini give the highest-resolution global views of Jupiter at visible and 5 μ m wavelengths during the Juno mission (similar 5 μ m resolution is achieved at some epochs with VLT/VISIR, Fletcher et al. 2018 ). This contextual information is helpful for interpreting spatial variation in ammonia mixing ratios derived from Juno MWR measurements. In one specific example, the imaging context on PJ 4 (Figure 9 ) reveals that Juno's sub-spacecraft track passed between convective storm cores in the SEB, where depleted ammonia concentration detected by the Juno MWR (Bellotti & Steffes 2017 ) indicates strong downwelling. Accurate placement of the Juno track with respect to the storm allows quantitative comparison with numerical models of convection such as Li & Ingersoll ( 2015 ). In addition to studying the convective process, the data set is well-suited to studies of jets, waves, vortices, hazes, clouds, and circulation.
The 53 day cadence driven by the Juno orbital period synchronizes a worldwide coordinated observational effort across a wide range of the spectrum, from UV data shown here and in Grodent et al. ( 2018 ), to data in the millimeter and radio regime (de Pater et al. 2019a , 2019b ). Our synchronized observations of the GRS (Figure 10 ) are able to distinguish between explanations of dark features within this iconic storm system (Sánchez-Lavega et al. 2018 ), showing that they are regions of reduced cloud opacity rather than regions with darker cloud particles.
A strong emphasis has been placed on providing HLSPs from the HST and Gemini data sets into the MAST archive. 19 The combined data set should support a wide range of unanticipated science investigations, enabled by its regular sampling at 53 day intervals over the multi-year duration of the Juno mission.
Based on observations obtained with NIRI at the Gemini Observatory, which is operated by the Association of Universities for Research in Astronomy, Inc. (AURA), under a cooperative agreement with the NSF on behalf of the international Gemini partnership: the National Science Foundation (United States), National Research Council (Canada), CONICYT (Chile), Ministerio de Ciencia, Tecnología e Innovación Productiva (Argentina), Ministério da Ciência, Tecnologia e Inovação (Brazil), and Korea Astronomy and Space Science Institute (Republic of Korea); on observations made with WFC3 on the NASA/ESA Hubble Space Telescope, obtained at the Space Telescope Science Institute, which is operated by AURA under NASA contract NAS 5-26555; on observations obtained with VLT/VISIR at the European Organisation for Astronomical Research in the Southern Hemisphere (under ESO programme 098.C-0681D).
This publication does not include data from the NASA Juno mission, other than MWR boresight pointings (at times of lightning sferic detections) that are publicly available as part of Brown et al. ( 2018 ), and timing and pointing data available from the Mission Juno public website ( https://missionjuno.swri.edu ).
Team members' contributions were supported by the Space Telescope Science Institute (for program numbers listed in Table 3 ), which is operated by AURA under NASA contract NAS 5-26555; by NASA under Cooperative Agreement 80NSSC19M0189, grant NNX16AP12H issued through the NASA Earth and Space Science Fellowship program, grants NNX14AJ43G and 80NSSC18K1001 issued through the Planetary Astronomy program, grant NNX16AP12H issued through the Earth and Space Science Fellowship program, and grant NNX15AJ41G issued through the Solar System Observations program; by the Gemini Observatory, which is operated by AURA on behalf of the international Gemini partnership; by NASA through the Juno Project; by NASA to the Jet Propulsion Laboratory, California Institute of Technology; by a Royal Society Research Fellowship; and by European Research Council Consolidator grant No. 723890 issued by the European Union's Horizon 2020 research and innovation programme.
This work was enabled by the location of the Gemini North telescope within the Maunakea Science Reserve, adjacent to the summit of Maunakea. We are grateful for the privilege of observing Ka'āwela (Jupiter) from a place that is unique in both its astronomical quality and its cultural significance.
Appendix: Additional Periodograms
Figure 6 presents periodogram analyses for two sets of data, as discussed in Section 5.1 . Table 6 lists several additional sets that were analyzed for periodicity, all of which are shown in this Appendix. For each periodogram shown in Figures 14 – 20 , the caption gives the subset of zonal wind measurements (Table 6 ) used in the analysis.
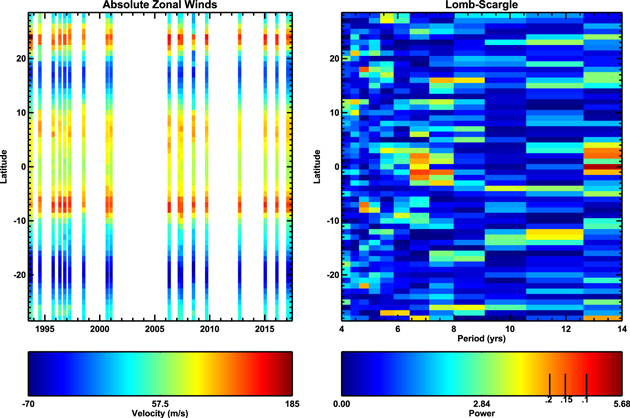
Figure 14. Zonal wind and periodogram data and colors as described in Figure 6 , for the "Tollefson et al. ( 2017 )" set in Table 6 .

Figure 15. Zonal wind and periodogram data and colors as described in Figure 6 , for the "Subset 2018" set in Table 6 .

Figure 16. Zonal wind and periodogram data and colors as described in Figure 6 , for the "HST only" set in Table 6 .
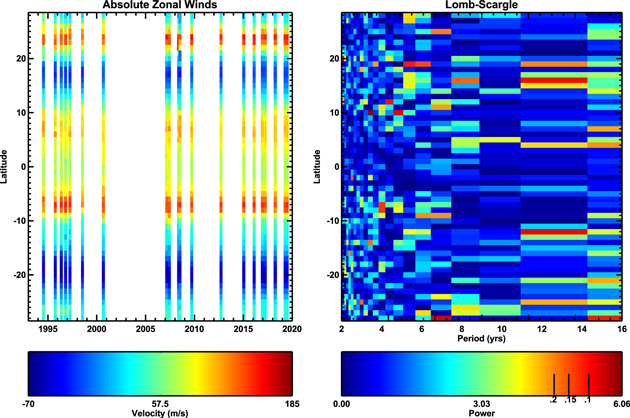
Figure 17. Zonal wind and periodogram data and colors as described in Figure 6 , for the "WFPC2 + WFC3" set in Table 6 .
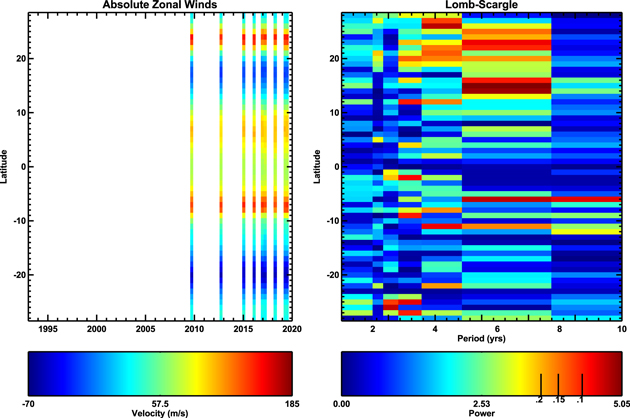
Figure 18. Zonal wind and periodogram data and colors as described in Figure 6 , for the "WFC3 only" set in Table 6 .
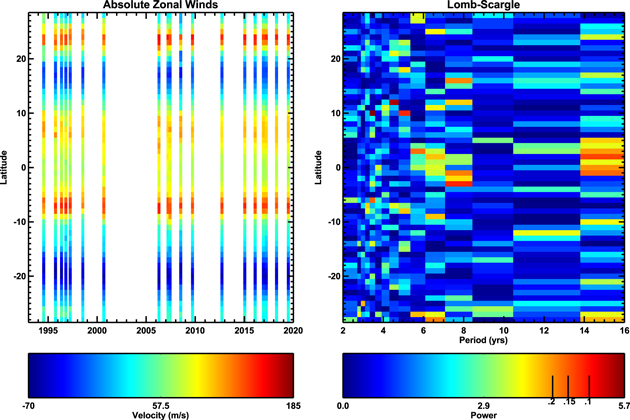
Figure 19. Zonal wind and periodogram data and colors as described in Figure 6 , for the "WFC3 only (filtered)" set in Table 6 .
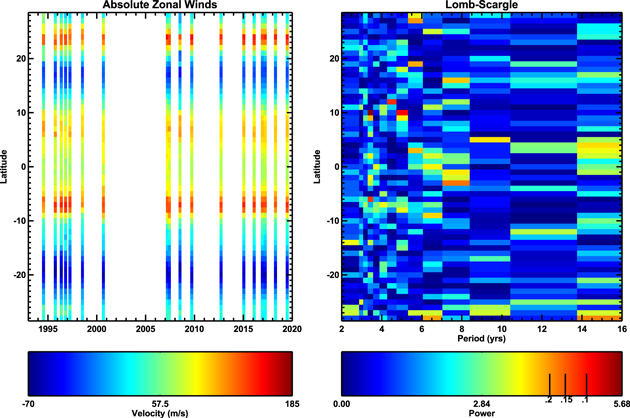
Figure 20. Zonal wind and periodogram data and colors as described in Figure 6 , for the "WFPC2 + WFC3 (filtered)" set in Table 6 .
AstroConda documentation (at the time of writing) is available at http://astroconda.readthedocs.io/ .
JPL Horizons URL is https://ssd.jpl.nasa.gov/?horizons .
WFC3/UVIS throughputs are at http://stsci.edu/~WFC3/UVIS/SystemThroughput or http://stsci.edu/hst/instrumentation/wfc3/performance/throughputs .
WFCJ HLSPs are available in doi: 10.17909/T94T1H .
OPAL HLSPs are available in Simon ( 2015 ).
TinyTim URL is http://tinytim.stsci.edu/cgi-bin/tinytimweb.cgi .
Versions of these profiles in planetocentric latitude coordinates are available on request.
WFCJ HLSPs are available in doi: 10.17909/T94T1H , and OPAL HLSPs are available in Simon ( 2015 ).
The atmosphere of Jupiter
- Published: March 1976
- Volume 18 , pages 603–639, ( 1976 )
Cite this article
- Andrew P. Ingersoll 1
127 Accesses
2 Citations
Explore all metrics
Current information on the neutral atmosphere of Jupiter is reviewed, with approximately equal emphasis on composition and thermal structure on the one hand, and markings and dynamics on the other. Studies based on Pioneer 10 and 11 data are used to refine the atmospheric model. Data on the interior are reviewed for the information they provide on the deep atmosphere. The markings and dynamics are discussed with emphasis on qualitative relationships and analogies with phenomena in the Earth's atmosphere.
This is a preview of subscription content, log in via an institution to check access.
Access this article
Subscribe and save.
- Get 10 units per month
- Download Article/Chapter or Ebook
- 1 Unit = 1 Article or 1 Chapter
- Cancel anytime
Price includes VAT (Russian Federation)
Instant access to the full article PDF.
Rent this article via DeepDyve
Institutional subscriptions
Similar content being viewed by others
Tenuous Atmospheres in the Solar System
Atmospheric Physics and Atmospheres of Solar-System Bodies
Aitken, D. K. and Jones, B.: 1972, Nature (London) 240 , 230.
Google Scholar
Anderson, J. D., Hubbard, W. B., and Slattery, W. L.: 1974a, Astrophys. J. 193 , L149.
Anderson, J. D., Null, G. W., and Wong, S. K.: 1974b, J. Geophys. Res. 79 , 3661.
Armstrong, K. R., Harper, D. A., Jr., and Low, F. J.: 1972, Astrophys. J. 178 , L89.
Aumann, H. H., Gillespie, C. M., Jr., and Low, F. J.: 1969, Astrophys. J. 157 , L69.
Baker, A. L., Baker, L. R., Beshore, E., Blenman, C., Castillo, N. D., Chen, Y.-P., Doose, L. R., Elston, J. P., Fountain, J. W., Gehreis, T., Kendall, J. H., KenKnight, C. E., Norden, R. A., Swindell, W., Tomasko, M. G., and Coffeen, D. L.: 1975, Science 188 , 468.
Barcilon, A. and Gierasch, P. J.: 1970, J. Atmospheric Sci. 27 , 550.
Bar-Nun, A.: 1975, Icarus 24 , 86.
Böhm, K.-H.: 1967, in R. N. Thomas (ed.), ‘Aerodynamic Phenomena in Stellar Atmospheres’, IAU Symp. 28 , 366.
Brinkmann, R. T.: 1974, Nature (London) 230 , 515.
Busse, F. H.: 1970, Astrophys. J. 159 , 629.
Busse, F. H.: 1975, Paper presented at the Jupiter Conference, Tucson, Arizona, May 19–23, 1975.
Cameron, A. G. W.: 1973, Space Sci. Rev. 14 , 392.
Carlson, R. W. and Judge, D. L.: 1974, J. Geophys. Res. 79 , 3623.
Carr, T. D.: 1971, Astrophys. Letters 7 , 157.
Chapman, C. R.: 1969, J. Atmospheric Sci. 26 , 986.
Chase, S. C., Ruiz, R. D., Münch, G., Neugebauer, G. Schroeder, M., and Trafton, L. M.: 1974, Science 183 , 315.
Divine, N.: 1971, ‘The Planet Jupiter (1970)’, NASA Space Vehicle Design Criteria (Environment), NASA SP-8069, Dec.
Duncombe, R. L., Kepczynski, W. J., and Seidelmann, P. K.: 1973, Fundamentals Cosmic Phys. 1 , 119.
Elliot, J., Wasserman, L., Veverka, J., Sagan, C., and Liller, W.: 1974, Astrophys. J. 190 , 719.
Flasar, F. M.: 1973, Astrophys. J. 186 , 1097.
French, R. G. and Gierasch, P. J.: 1974, J. Atmospheric Sci. 31 , 1707.
Gierasch, P. J.: 1973, Icarus 19 , 482.
Gierasch, P. J. and Goody, R. M.: 1969, J. Atmospheric Sci. 26 , 979.
Gierasch, P. J., Ingersoll, A. P., and Williams, R. T.: 1973, Icarus 19 , 473.
Gillett, F. C. and Westphal, J. A.: 1973, Astrophys. J. 179 , L153.
Gillett, F. C., Low, F. J., and Stein, W. A.: 1969, Astrophys. J. 157 , 925.
Graboske, H. C., Jr., Pollack, J. B., Grossman, A. S., and Olness, R. J.: 1975, Astrophys. J. , in press.
Gulkis, S.: 1973, Space Sci. Rev. 14 , 497.
Gulkis, S. and Poynter, R.: 1972, Phys. Earth Planetary Interiors 6 , 36.
Gulkis, S., Klein, M. J., and Poynter, R. L.: 1974, in A. Woszeryk and C. Iwaniszewska (eds.), ‘Exploration of the Planetary System’, IAU Symp. 65 , 367.
Henry, R. J. W. and McElroy, M. B.: 1969, J. Atmospheric Sci. 26 , 912.
Hess, S. L. and Panofsky, A. A.: 1951, in Compedium of Meteorology , American Meteorological Society, Boston, p. 391.
Hide, R.: 1961, Nature (London) 190 , 895.
Hide, R.: 1963, Mem. Soc. Roy. Sci. Liège (Series) V VII , 481.
Hide, R.: 1966, Planetary Space Sci. 14 , 669.
Houck, J., Pollack, J. B., Schack, D., and Reed, R.: 1974, Amer. Astron. Soc., 5th Annual Meeting, Palo Alto, Ca.
Howard, H. T., Tyler, G. L., Fjeldbo, G., Kliore, A. J., Levy, G. S., Brunn, D. L., Dickinson, R., Edelson, R. E., Martin, W. L., Postal R. B., Seidel, B., Sesplaukis, T. T., Shirley, D. L., Stelzried, C. T., Sweetnam, D. N., Zygielbaum, A. I., Esposito, P. B., Anderson, J. D., Shaprio, I. I., and Reasenberg, R. D.: 1974, Science 183 , 1298.
Hubbard, W. B.: 1969, Astrophys. J. 155 , 333.
Hubbard, W. B.: 1970, Astrophys. J. 162 , 687.
Hubbard, W. B.: 1973, Space Sci. Rev. 14 , 424.
Hubbard W. B. and Smoluchowski R.: 1973, Space Sci. Rev. 14 , 599.
Hubbard, W. B., and VanFlandern, T. C.: 1972, Astron. J. 77 , 65.
Hubbard, W. B., Hunten, D. M., and Kliore, A.: 1975, Astrophys. J. , in press.
Hubbard, W. B., Nather, R. E., Evans, D. S., Tull, R. G., Wells, D. C., van Citters, G. W., Warner, B., and Vanden Bout, P.: 1972, Astron. J. 77 , 41.
Hubbard, W. B., Trubitsyn, V. P., and Zharkov, V. N.: 1974, Icarus 21 , 147.
Hunten, D. M. and Münch, G.: 1973, Space Sci. Rev. 14 , 433.
Ingersoll, A. P.: 1973, Science 182 , 1346.
Ingersoll, A. P.: 1975, Paper presented at Jupiter Conference, Tucson, Arizona, May 19–23, 1975.
Ingersoll, A. P. and Cuzzi, J. N.: 1969, J. Atmospheric Sci. 26 , 981.
Ingersoll, A. P., Münch, G., Neugebauer, G., Diner, D. J., Orton, G. S., Schupler, B., Schroeder, M., Chase, S. C., Ruiz, R. D., and Trafton, L. M.: 1975a, Science 188 , 472.
Ingersoll, A. P., Münch, G., Neugebauer, G., and Orton, G. S.: 1975b, Paper presented at Jupiter Conference, Tucson, Arizona, May 19–23, 1975.
Keay, C. S. L., Low, F. J., Rieke, G. H., and Minton, R. B.: 1973, Astrophys. J. 183 , 1063.
Khare, B. N. and Sagan, C.: 1973, Icarus 20 , 311.
Kieffer, H. H.: 1967, J. Geophys. Res. 72 , 3179.
Kliore, A., Fjeldbo, G., Seidel, B. L., Sesplankis, T. T., Sweetnam, D. W., Woiceshyn, P. M.: 1975, Science 188 , 474.
Larson, H. P., Fink, U., Treffers, R., Gautier, T. N.: 1975, Astrophys. J. , in press.
Lewis, J. S.: 1969, Icarus 10 , 365.
Lewis, J. S.: 1973, Space Sci. Rev. 14 , 401.
Lewis, J. S. and Prinn, R. G.: 1970, Science 169 , 472.
Maxworthy, T. and Redekopp, L. G.: 1975, Paper presented at Jupiter Conference, Tucson, Arizona, May 19–23, 1975.
McElroy, M. B.: 1973, Space Sci. Rev. 14 , 460.
Molton, P. M. and Ponnamperuma, C.: 1974, Icarus 21 , 166.
Murphy, R. E. and Fesen, R. A.: 1974, Icarus 21 , 42.
Newburn, R. L., Jr. and Gulkis, S.: 1973, Space Sci. Rev. 14 , 179.
Newburn, R. L., Jr. and Gulkis, S.: 1975, in Foundations of Space Biology and Medicine Vol. I , part 2, ch. 3, NASA Office of Life Science, Washington, D. C.
Ohring, G.: 1973, Astrophys. J. 184 , 1027.
Orton, G. S.: 1975a, Icarus , in press.
Orton, G. S.: 1975b, Paper presented at Jupiter Conference, Tucson, Arizona, May 19–23, 1975.
Orton, G. S.: 1975c, Icarus , in press.
Orton, G. S.: 1975d, Icarus , in press.
Owen, T. and Mason, H. P.: 1969, J. Atmospheric Sci. 26 , 870.
Palmén, E. and Newton, C. W.: 1969, Atmospheric Circulation Systems , Academic Press, New York and London.
Peek, B. M.: 1958, The Planet Jupiter , Faber and Faber, London.
Pilcher, S. B., Prinn, R. B., and McCord, T. B.: 1973, J. Atmospheric Sci. 30 , 302.
Podolak, M. and Cameron, A. G. W.: 1974, Icarus 22 , 123.
Pollack, J. B. and Reynolds, R. T.: 1974, Icarus 21 , 248.
Pollard. D. and Ingersoll, A. P.: 1975, to be published.
Prinn, R. G.: 1970, Icarus 13 , 424.
Prinn, R. G. and Lewis, J. S.: 1975, Science 190 , 274.
Reese, E. J. and Smith, B. A.: 1968, Icarus 9 , 474.
Ridgway, S. T.: 1974, Astrophys. J. 187 , L41.
Roberts, P. H.: 1968, Phil. Trans. Roy. Soc. London A263 , 93.
Salpeter, E. E.: 1973, Astrophys. J. 181 , L83.
Savage, B. D. and Danielson, R. E.: 1968, in P. J. Brancazio and A. G. W. Cameron (eds.), Infrared Astronomy , Gordon and Breach, New York, p. 211.
Smith, B. A.: 1975, Paper presented at Jupiter Conference, Tucson, Arizona, May 19–23, 1975.
Smoluchowski, R.: 1973, Astrophys. J. 185 , L95.
Solberg, H. G., Jr.: 1969, Planetary Space Sci. 17 , 1573.
Spiegel, E. N.: 1963, Astrophys. J. 138 , 216.
Stauffer, D. and Kiang, C. S.: 1974, Icarus 21 , 129.
Stone, P. H.: 1967, J. Atmospheric Sci. 24 , 642.
Stone, P. H.: 1971, Geophys. Fluid Dyn. 2 , 147.
Stone, P. H.: 1972, J. Atmospheric Sci. 29 , 405.
Stone, P. H.: 1974, J. Atmospheric Sci. 31 , 1471.
Streett, W. B.: 1973, Astrophys. J. 186 , 1107.
Streett, W. B., Ringermacher, H. I., and Veronis, G.: 1971, Icarus 14 , 319.
Strobel, D. F.: 1973, J. Atmospheric Sci. 30 , 489.
Strobel, D. F.: 1974, Astrophys. J. 192 , L47.
Strobel, D. F. and Smith, G. R.: 1973, J. Atmospheric Sci. 30 , 718, 964 (corrigendum).
Swindell, W. and Doose, L. R.: 1974, J. Geophys. Res. 79 , 3634.
Taylor, D. J.: 1965, Icarus 4 362.
Taylor, F. W.: 1973, J. Atmospheric Sci. 30 , 677.
Terrile, R. J. and Westphal, J. A.: 1975, Amer. Astron. Soc. Div. Planetary Sci., 6th Annual Meeting, Columbia, Md.
Tomasko, M. G.: 1974, Astrophys. J. 187 , 641.
Tomasko, M. G., Clements, A. E., and Castillo, N. D.: 1974, J. Geophys. Res. 79 , 3653.
Trafton, L. M.: 1967, Astrophys. J. 147 , 765.
Trafton, L. M.: 1973, Astrophys. J. 179 , 971.
Trafton, L. M. and Stone, P. H.: 1974, Astrophys. J. 188 , 649.
Vapillion, L., Combes, M., and Lecacheux, J.: 1974, Astron. Astrophys. 29 135.
Veverka, J., Wasserman, L. H., Elliot, J., Sagan, C., and Liller, W.: 1974, Astron. J. 79 , 74.
Wallace, L., Caldwell, J. J., and Savage, B. D.: 1972, Astrophys. J. 172 , 755.
Wallace, L., Prather, M., and Belton, M. J. S.: 1974, Astrophys. J. 193 , 481.
Wasserman, L. H.: 1974, Icarus 22 , 105.
Weidenschilling, S. J. and Lewis, J. S.: 1973, Icarus 20 , 465.
Westphal, J. A., Matthews, K., and Terrile, R. J.: 1974, Astrophys. J. 188 , L111.
Williams, G. P. and Robinson, J. B.: 1973, J. Atmospheric Sci. 30 , 684.
Download references
Author information
Authors and affiliations.
Division of Geological and Planetary Sciences, California Institute of Technology, 91125, Pasadena, Calif., U.S.A
Andrew P. Ingersoll
You can also search for this author in PubMed Google Scholar
Additional information
Contribution No. 2652 of the Division of Geological and Planetary Sciences, California Institute of Technology, Pasadena, Calif. 91125, U.S.A.
Rights and permissions
Reprints and permissions
About this article
Ingersoll, A.P. The atmosphere of Jupiter. Space Sci Rev 18 , 603–639 (1976). https://doi.org/10.1007/BF00169519
Download citation
Received : 28 July 1975
Issue Date : March 1976
DOI : https://doi.org/10.1007/BF00169519
Share this article
Anyone you share the following link with will be able to read this content:
Sorry, a shareable link is not currently available for this article.
Provided by the Springer Nature SharedIt content-sharing initiative
- Atmospheric Model
- Thermal Structure
- Current Information
- Neutral Atmosphere
- Find a journal
- Publish with us
- Track your research

Jupiter Exploration
While Jupiter has been known since ancient times, the first detailed observations of this planet were made by Galileo Galilei in 1610 with a small, homemade telescope. More recently, this planet has been visited by orbiters, probes, and by spacecraft passing by on their way to other worlds. NASA's Pioneer 10 and 11, and Voyager 1 and 2 were the first to fly by Jupiter in the 1970s. Later, the Galileo spacecraft orbited the gas giant for almost eight years, and dropped a probe into its atmosphere. Cassini took detailed photos of Jupiter on its way to neighboring Saturn, as did New Horizons on its journey to Pluto and the Kuiper Belt. NASA’s Juno spacecraft has been studying Jupiter from orbit since July 2016. Europa Clipper will launch in October 2024 to study Jupiter's icy moon, Europa.
Past missions
Missions en route.
ESA's Juice Spacecraft
Future Missions
Europa clipper, missions to jupiter.
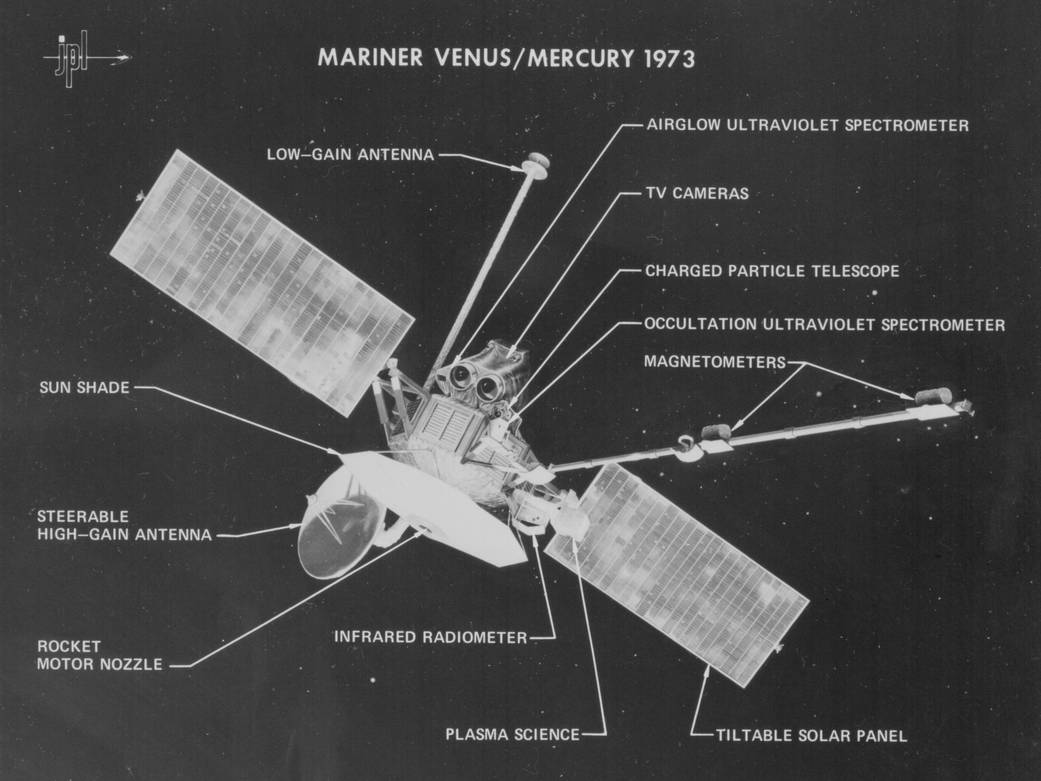
NASA's first spacecraft to visit the outer planets, Pioneer 10 was designed as a 21-month mission to Jupiter, yet lasted more than 30 years. After its Jupiter encounter in 1973, it continued beyond the solar system, sending its last signal to Earth in January 2003 from a distance of 7.6 billion miles.
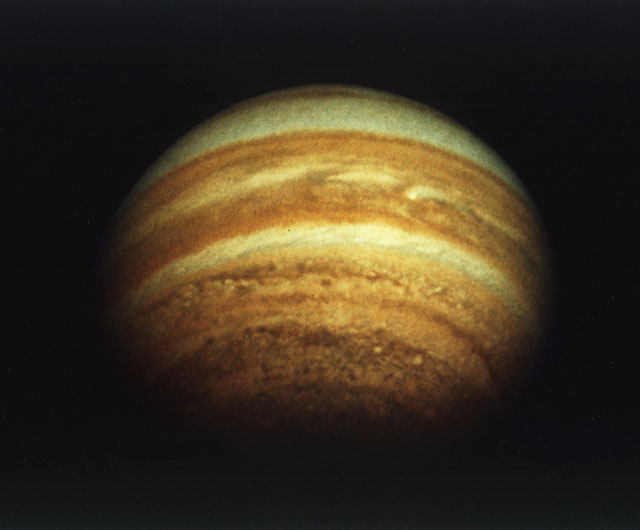
The sister spacecraft to Pioneer 10, it flew even closer to Jupiter in 1974 than its sibling had, passing while en route to its destination, Saturn. After studying the ringed planet, Pioneer 11 exited the solar system, and like its sibling carries a plaque with a message for any intelligent beings that may encounter it.
As it flew by Jupiter in March 1979, Voyager 1 discovered a thin ring around the planet, two new moons, and active volcanoes on the volatile moon Io, before continuing on to Saturn and interstellar space.
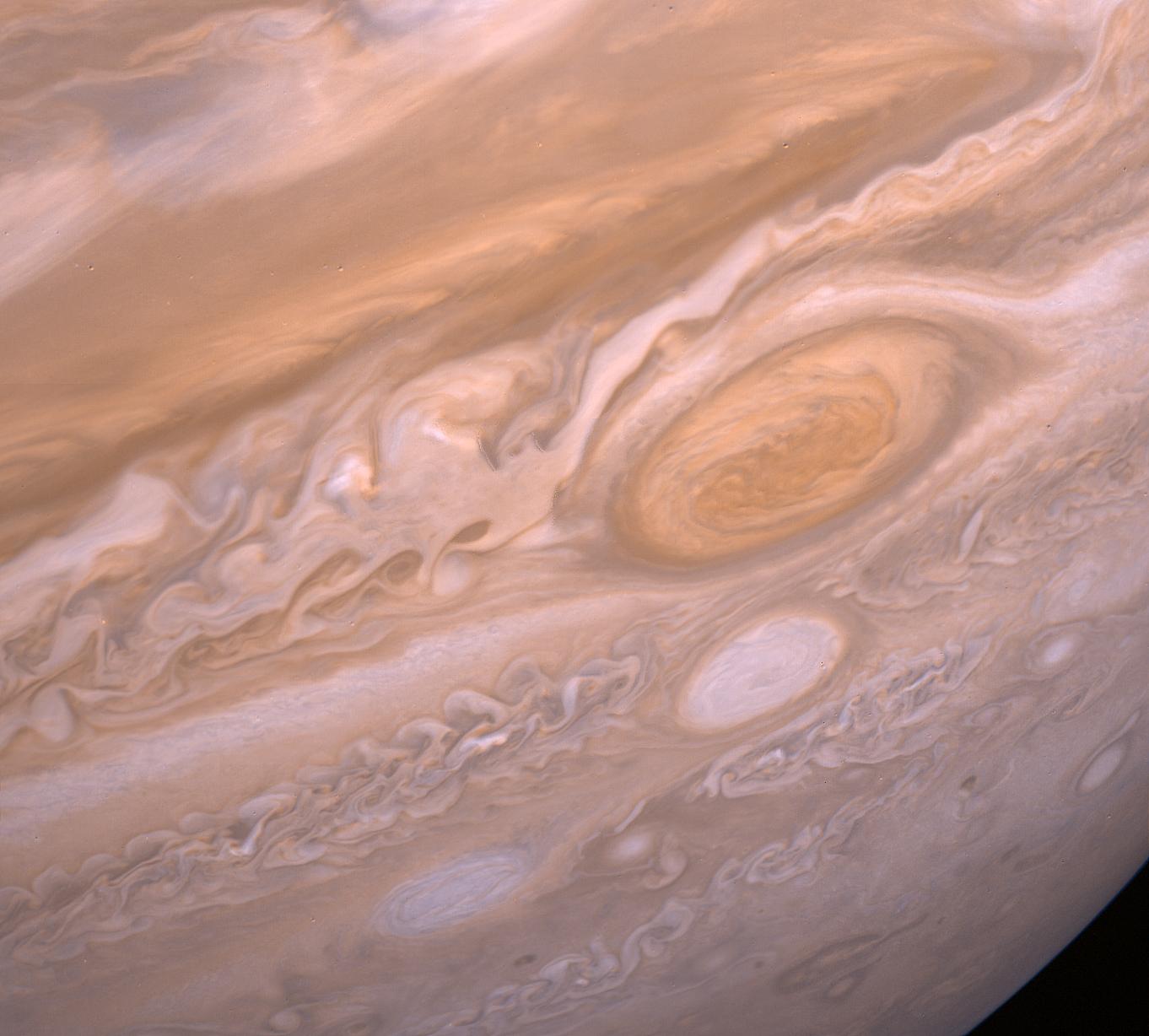
Voyager 2 began transmitting images of Jupiter April 24, 1979, for time-lapse movies of atmospheric circulation. Unlike Voyager 1, Voyager 2 made close passes to the Jovian moons on its way into the system, with scientists especially interested in more information from Europa and Io.
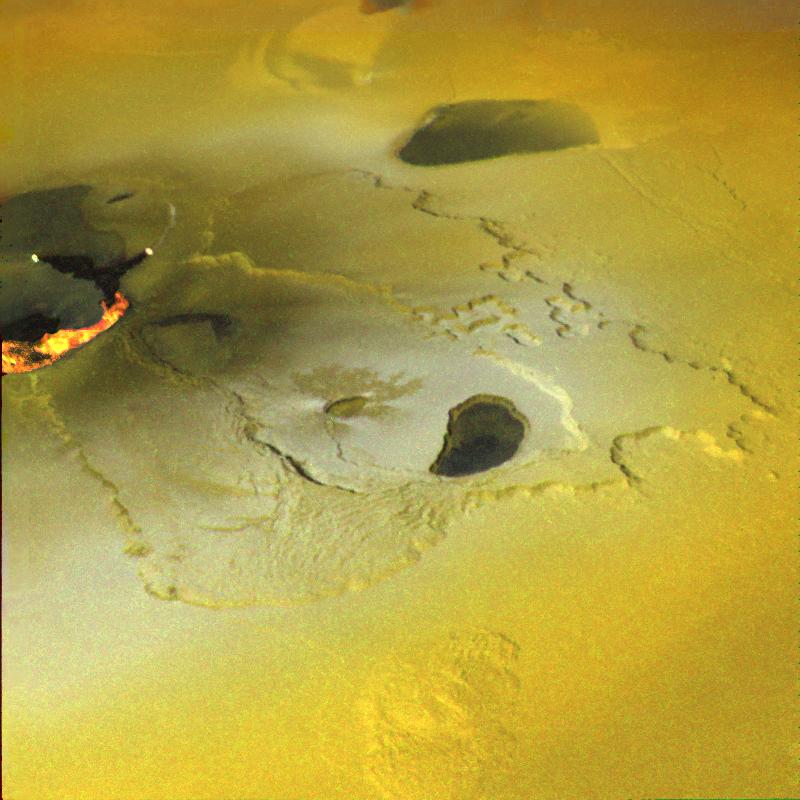
During Galileo's 34 orbits of Jupiter, it gathered unprecedented data on the planet's atmosphere, magnetosphere, and evolution. It observed a comet crash into the planet, and also deployed a probe – the first spacecraft to enter Jupiter's atmosphere. And while studying Jupiter's moons, Galileo discovered evidence of a subsurface ocean on Europa, Ganymede's unique magnetic field, and unparalleled volcanic activity on Io.
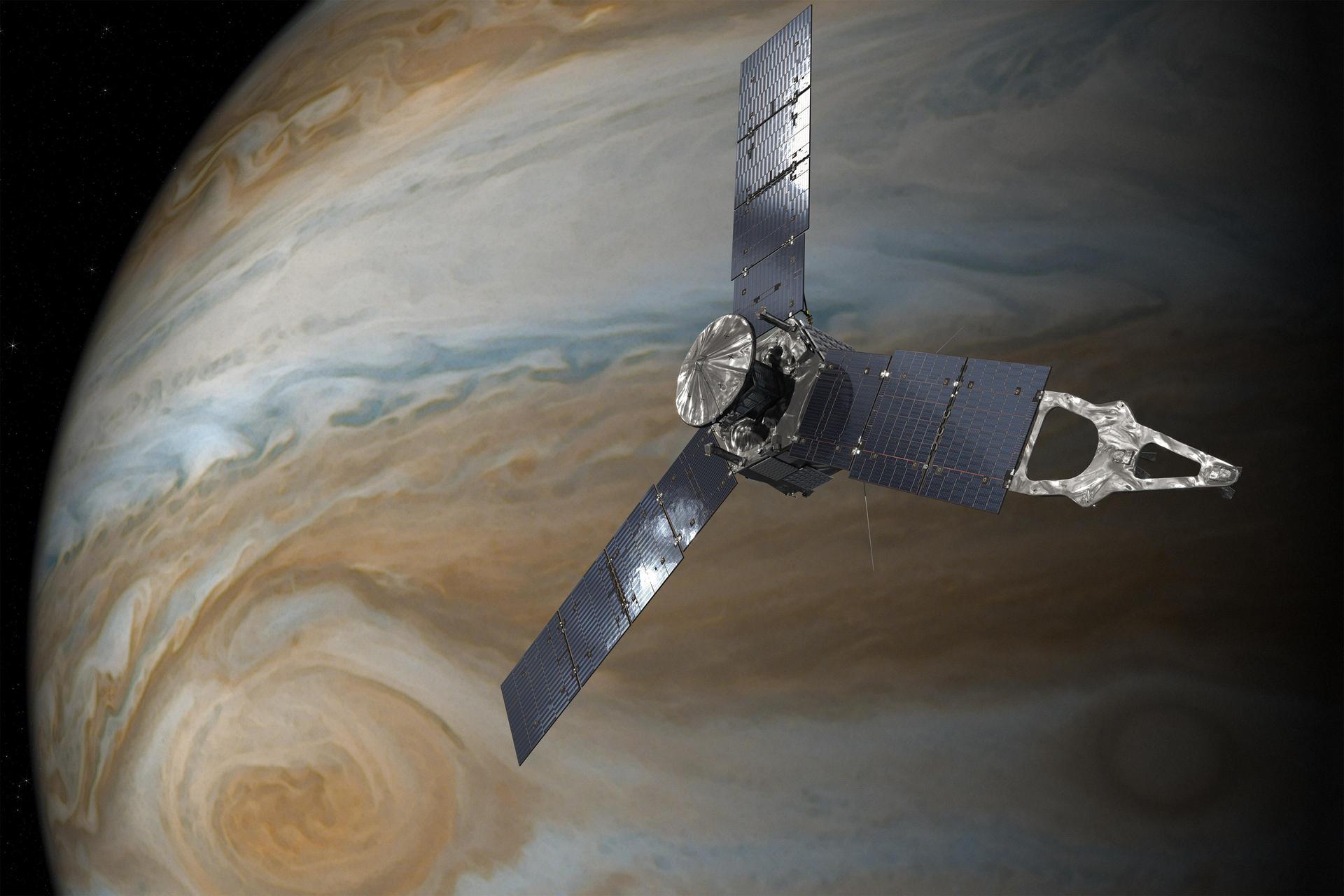
Juno has been In orbit around Jupiter since 2016. It's probing beneath the planet's dense clouds to answer questions about its origin and evolution, and is scheduled to continue investigating the solar system’s largest planet, its moons, faint rings, and surrounding environment through September 2025.
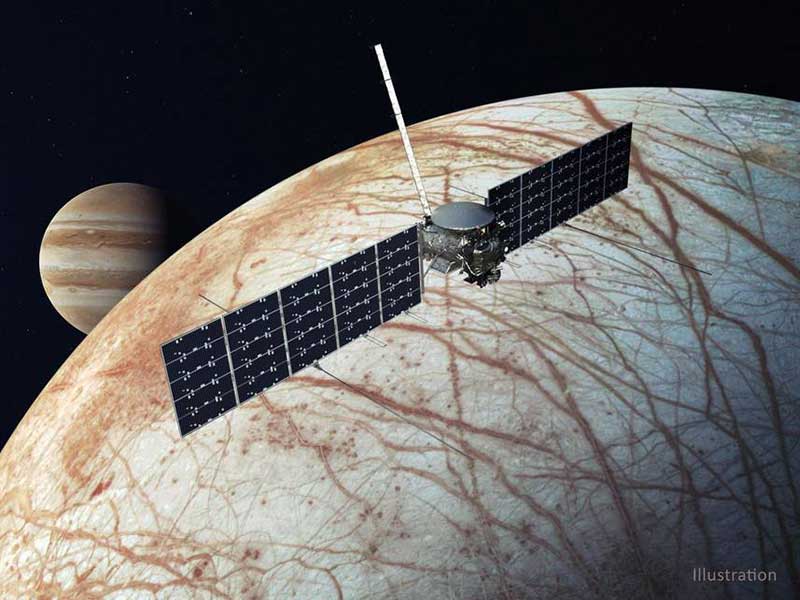
NASA's Europa Clipper is scheduled to launch in October 2024 and reach the planet in 2030. During dozens of flybys, the spacecraft will investigate Jupiter's icy moon Europa to determine whether there are places below the surface that could support life.
Discover More Topics From NASA
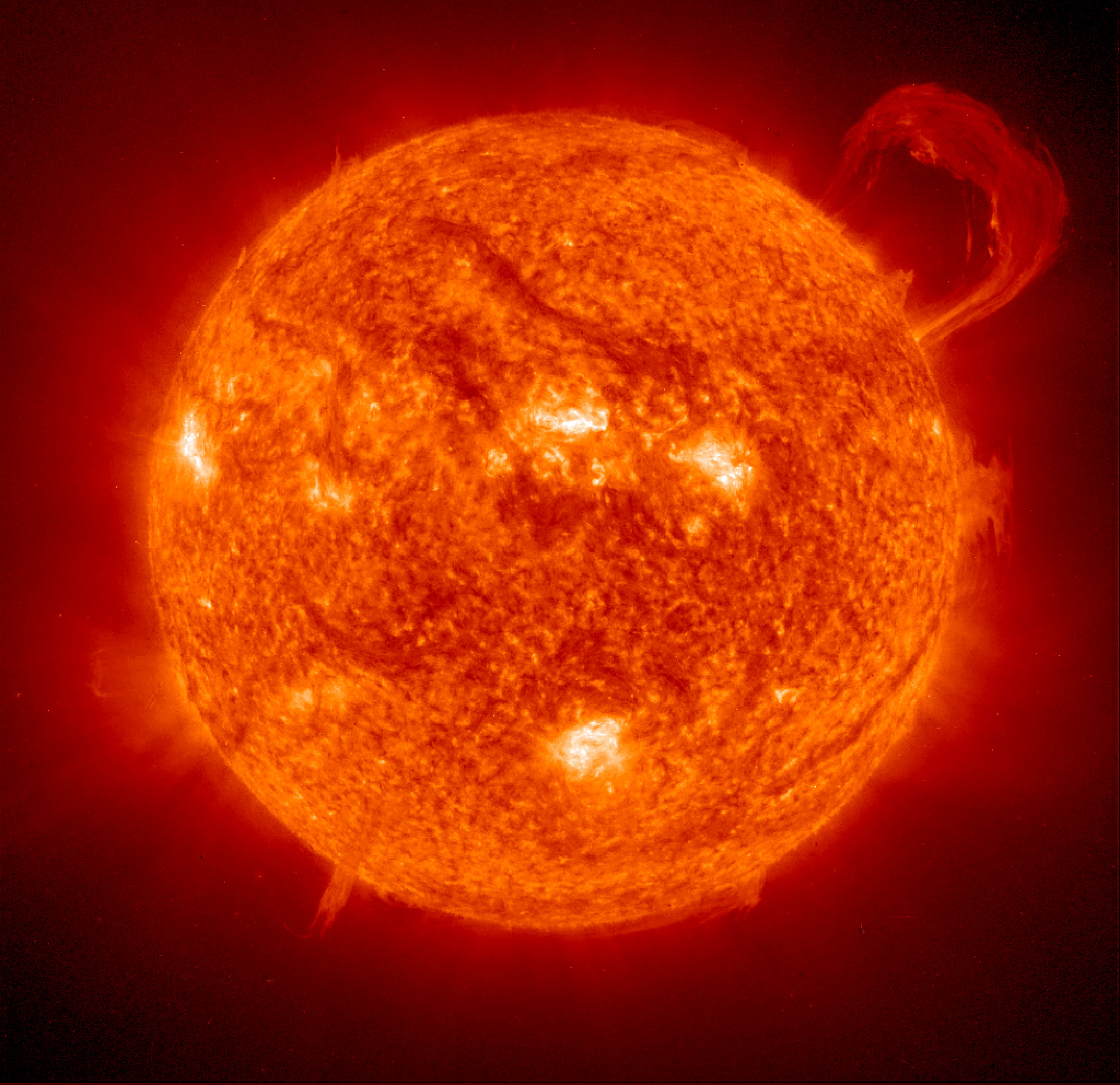
Asteroids, Comets & Meteors
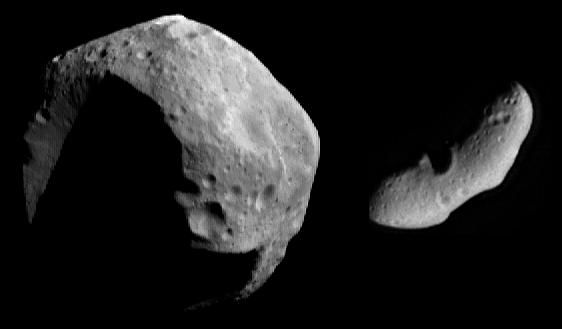
Kuiper Belt
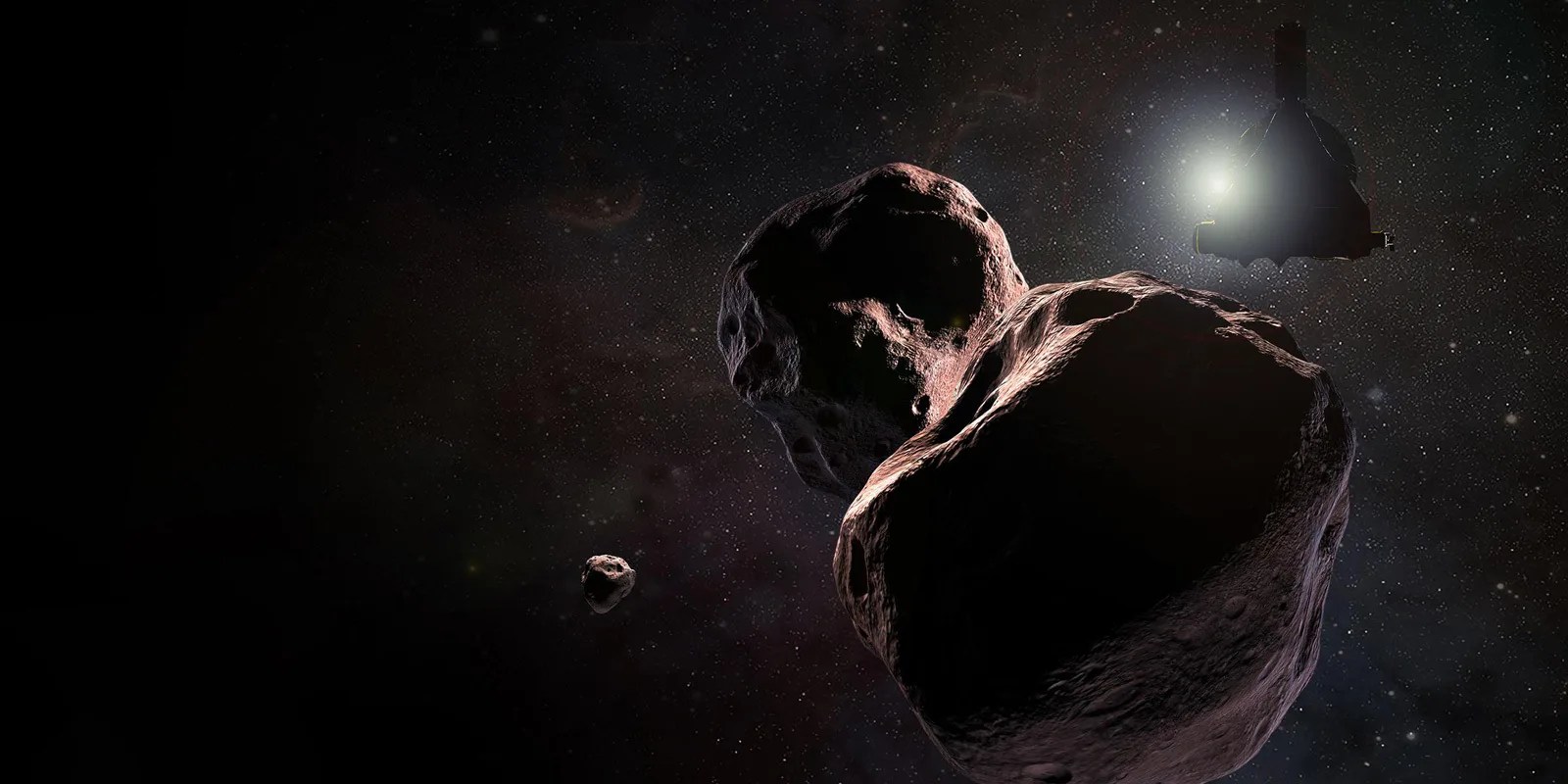
Search form
A new explanation for jupiter’s great, shrinking ‘spot’.

Hubble image of Jupiter. [Credit: NASA, ESA, A. Simon (Goddard Space Flight Center) and M.H. Wong (University of California, Berkeley)]
Jupiter’s Great Red Spot — the biggest windstorm in the solar system — is shrinking, and a new study may help explain why.
Located in Jupiter’s southern hemisphere, the Great Red Spot is a swirling, red-orange oval of high pressure more than 10,000 miles wide. It consistently blows more than 200 miles per hour in a counterclockwise direction, making it technically an anticyclone.
And it has been shrinking for the better part of a century, particularly over the past 50 years. While its latitudinal extent has remained relatively consistent, its longitudinal extent has contracted from 40 degrees in the late 19 th century to 14 degrees in 2016, when NASA’s Juno spacecraft arrived at the planet for a series of orbits.
“ Many people have looked at the Great Red Spot over the last 200 years and were as fascinated by it as I am,” said Caleb Keaveney, a Ph.D. student in Yale’s Graduate School of Arts and Sciences and lead author of a new study in the journal Icarus . “A lot of those people were not professional astronomers — they were just passionate and curious. That, plus the curiosity I see in people when I talk about my work, makes me feel like part of something bigger than myself.”
Some of the curiosity relating to the Great Red Spot has to do with the many mysteries that surround it, despite the fact it that it has been studied extensively. Astronomers don’t know precisely when the spot formed, why it formed, or even why it is red.
For the study, Keaveney, who is part of Yale’s Department of Earth & Planetary Sciences, and his co-authors, Gary Lackmann of North Carolina State University and Timothy Dowling of the University of Louisville, focused on the influence of smaller, transient storms on the Great Red Spot.
The researchers conducted a series of 3D simulations of the spot using the Explicit Planetary Isentropic-Coordinate (EPIC) model, an atmospheric model for planetary applications developed by Dowling in the 1990s. Some of these simulated interactions between the Great Red Spot and smaller storms of varying frequency and intensity, while another group of control simulations left out the small storms.
A comparison of the simulations suggested that the presence of other storms strengthened the Great Red Spot, causing the spot to grow larger.
“ We found through numerical simulations that by feeding the Great Red Spot a diet of smaller storms, as has been known to occur on Jupiter, we could modulate its size,” Keaveney said.
In part, the researchers based their modeling on long-lived high-pressure systems observed closer to home, in Earth’s atmosphere. These systems — known as “heat domes,” or “blocks” — occur regularly in the westerly jet streams that circulate across Earth’s mid-latitudes and play a major role in extreme weather events such as heat waves and droughts.
The longevity of these “blocks” has been linked to interactions with smaller, transient weather mechanisms, including high pressure eddies and anticyclones.
“ Our study has compelling implications for weather events on Earth,” Keaveney said. “Interactions with nearby weather systems have been shown to sustain and amplify heat domes, which motivated our hypothesis that similar interactions on Jupiter could sustain the Great Red Spot. In validating that hypothesis, we provide additional support to this understanding of heat domes on Earth.”
Keaveney said additional modeling will allow researchers to refine the new findings — and perhaps shed light on the Great Red Spot’s initial formation.
Funding for the research came from a North Carolina Space Grant and was supported by the Astronaut Scholarship Foundation and the Goldwater Scholarship Foundation. The research was conducted as an undergraduate research project in the Department of Marine, Earth, and Atmospheric Sciences at North Carolina State University.
Science & Technology
Media Contact
Michael Greenwood: [email protected] , 203-737-5151

Allez-y! Yale rowers go for gold at Paris Olympics

A robot that survives through self-amputation

Beinecke exhibition ‘lifts up’ the voices of Holocaust survivors

In Memoriam
James Scott, pathbreaking scholar in the social sciences
- Show More Articles
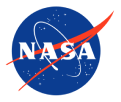
Suggested Searches
- Climate Change
- Expedition 64
- Mars perseverance
- SpaceX Crew-2
- International Space Station
- View All Topics A-Z
Humans in Space
Earth & climate, the solar system, the universe, aeronautics, learning resources, news & events.
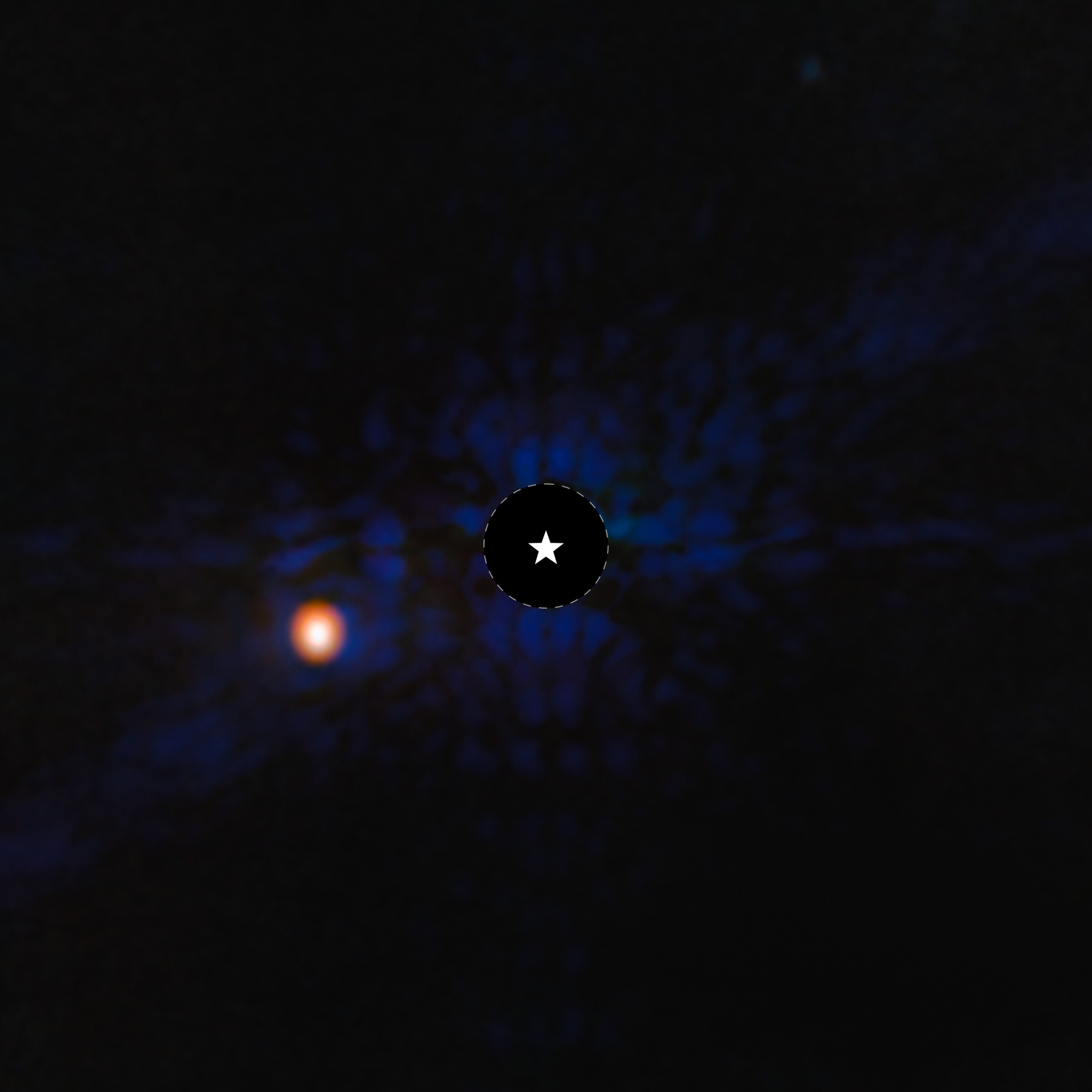
NASA’s Webb Images Cold Exoplanet 12 Light-Years Away
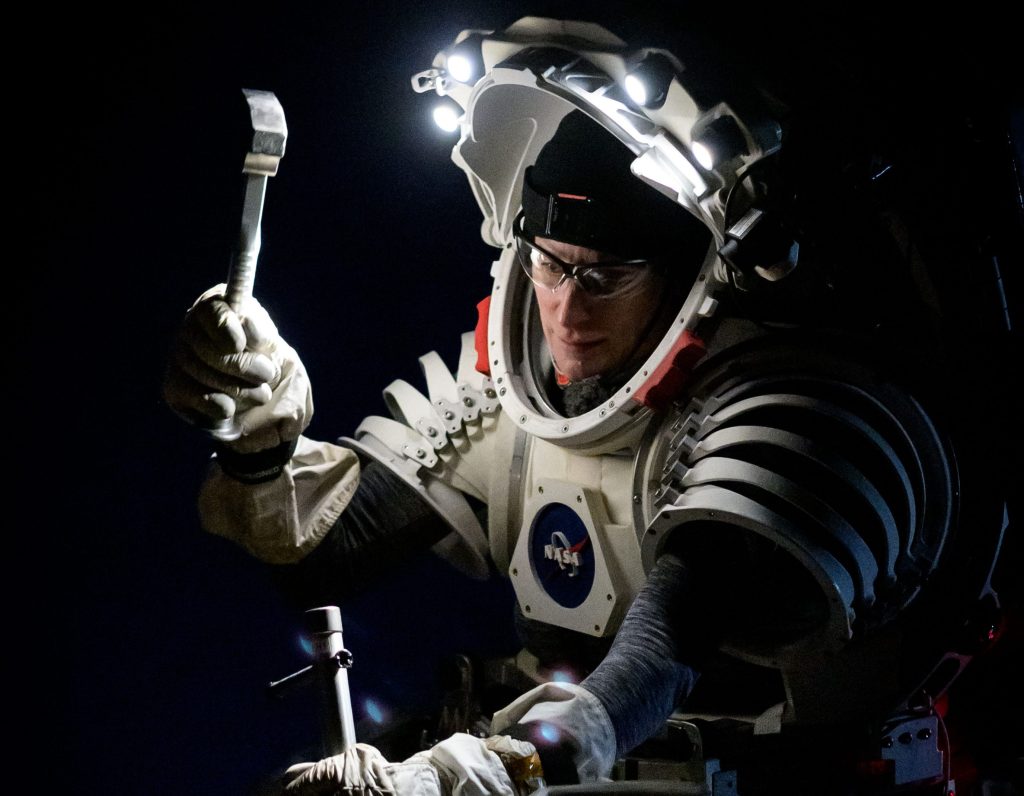
NASA Releases First Integrated Ranking of Civil Space Challenges

25 Years On, Chandra Highlights Legacy of NASA Engineering Ingenuity
- Search All NASA Missions
- A to Z List of Missions
- Upcoming Launches and Landings
- Spaceships and Rockets
- Communicating with Missions
- James Webb Space Telescope
- Hubble Space Telescope
- Why Go to Space
- Commercial Space
- Destinations
- Living in Space
- Explore Earth Science
- Earth, Our Planet
- Earth Science in Action
- Earth Multimedia
- Earth Science Researchers
- Pluto & Dwarf Planets
- Asteroids, Comets & Meteors
- The Kuiper Belt
- The Oort Cloud
- Skywatching
- The Search for Life in the Universe
- Black Holes
- The Big Bang
- Dark Energy & Dark Matter
- Earth Science
- Planetary Science
- Astrophysics & Space Science
- The Sun & Heliophysics
- Biological & Physical Sciences
- Lunar Science
- Citizen Science
- Astromaterials
- Aeronautics Research
- Human Space Travel Research
- Science in the Air
- NASA Aircraft
- Flight Innovation
- Supersonic Flight
- Air Traffic Solutions
- Green Aviation Tech
- Drones & You
- Technology Transfer & Spinoffs
- Space Travel Technology
- Technology Living in Space
- Manufacturing and Materials
- Science Instruments
- For Kids and Students
- For Educators
- For Colleges and Universities
- For Professionals
- Science for Everyone
- Requests for Exhibits, Artifacts, or Speakers
- STEM Engagement at NASA
- NASA's Impacts
- Centers and Facilities
- Directorates
- Organizations
- People of NASA
- Internships
- Our History
- Doing Business with NASA
- Get Involved
NASA en Español
- Aeronáutica
- Ciencias Terrestres
- Sistema Solar
- All NASA News
- Video Series on NASA+
- Newsletters
- Social Media
- Media Resources
- Upcoming Launches & Landings
- Virtual Events
- Sounds and Ringtones
- Interactives
- STEM Multimedia
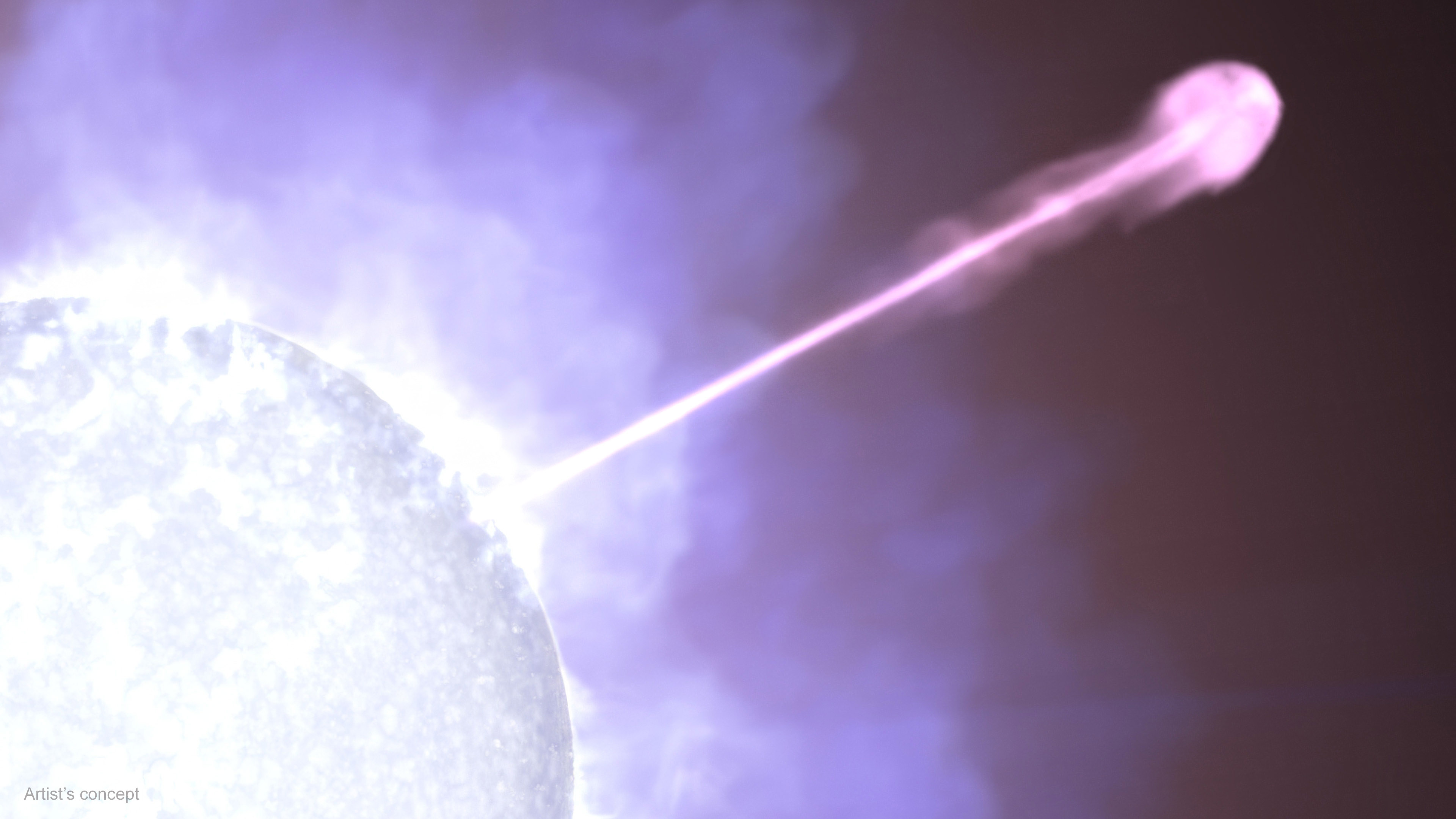
NASA’s Fermi Finds New Feature in Brightest Gamma-Ray Burst Yet Seen
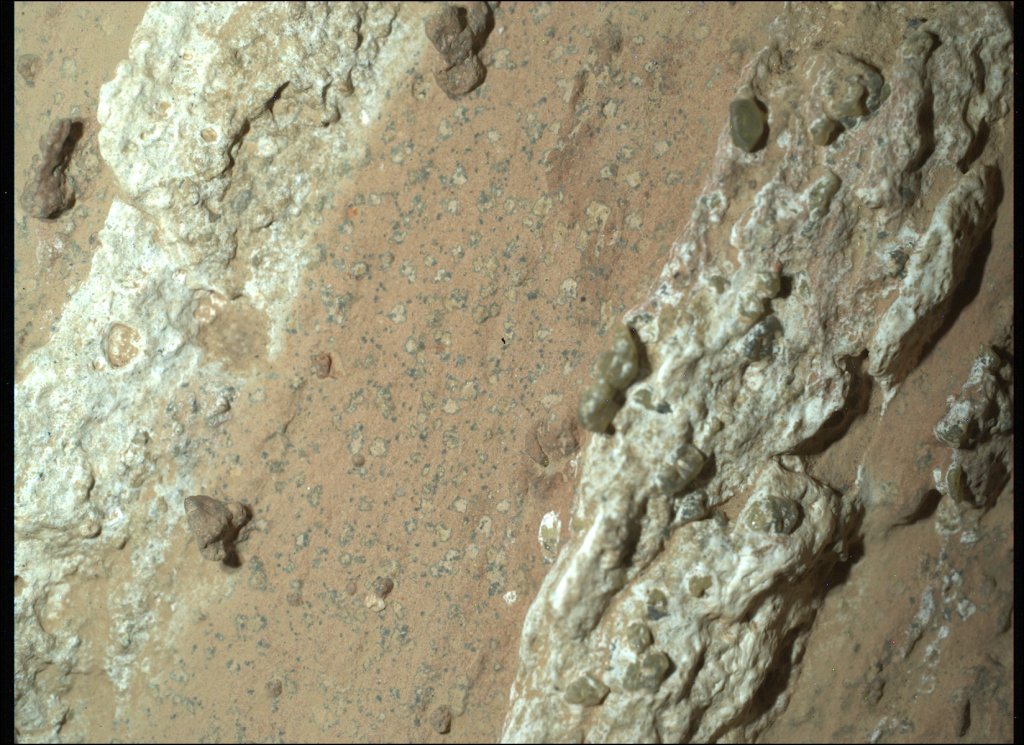
NASA’s Perseverance Rover Scientists Find Intriguing Mars Rock
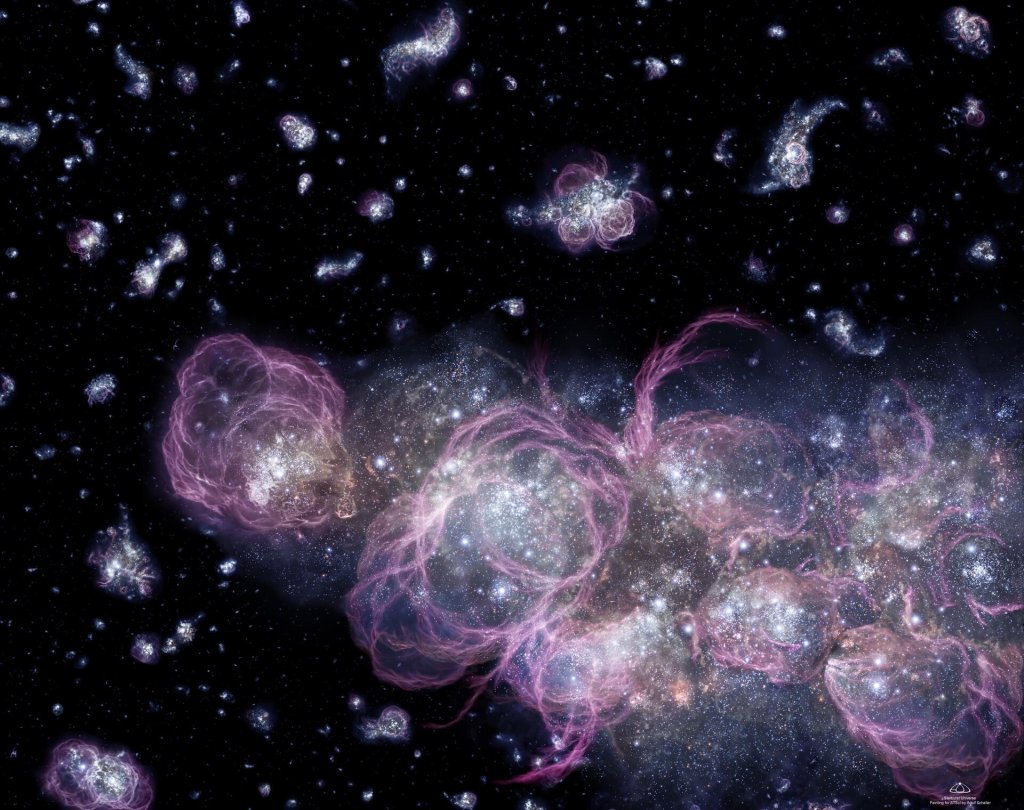
How NASA’s Roman Space Telescope Will Illuminate Cosmic Dawn

NASA Supports Burst Test for Orbital Reef Commercial Space Station
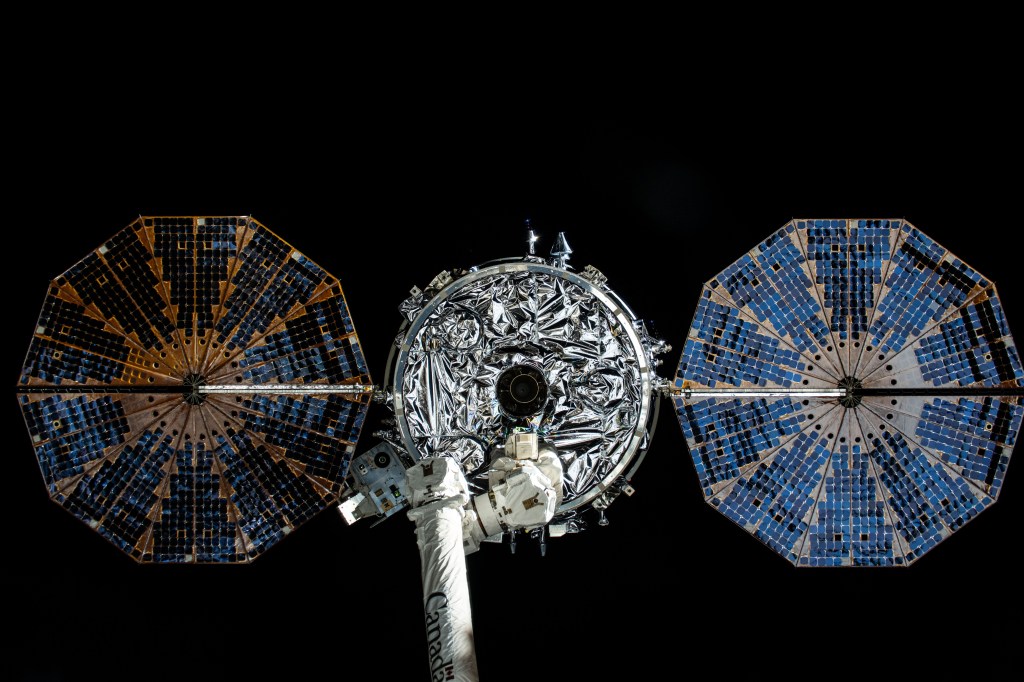
NASA’s 21st Northrop Grumman Mission Launches Scientific Studies to Station

Eileen Collins Broke Barriers as America’s First Female Space Shuttle Commander
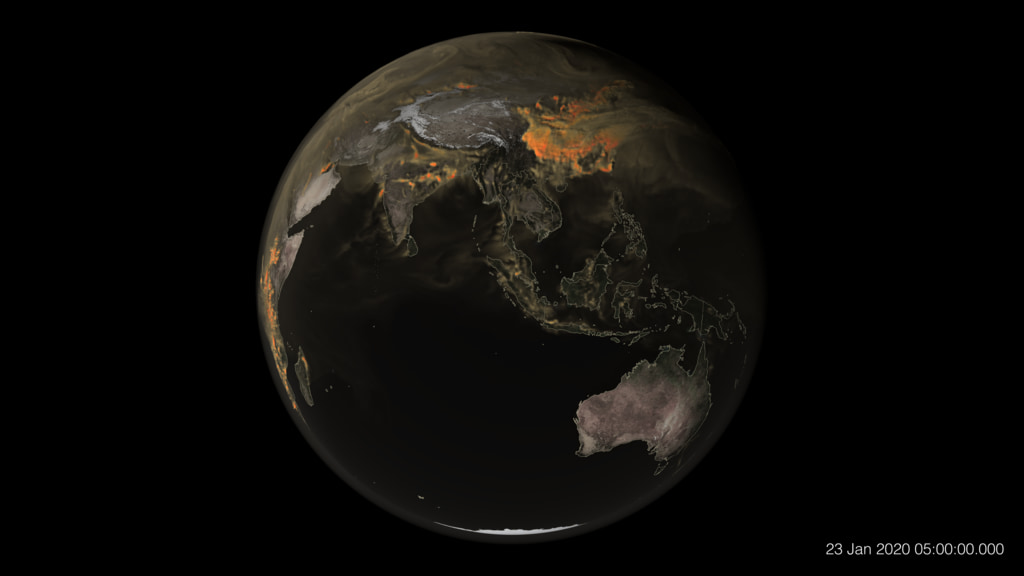
Watch Carbon Dioxide Move Through Earth’s Atmosphere

NASA-Funded Studies Explain How Climate Is Changing Earth’s Rotation
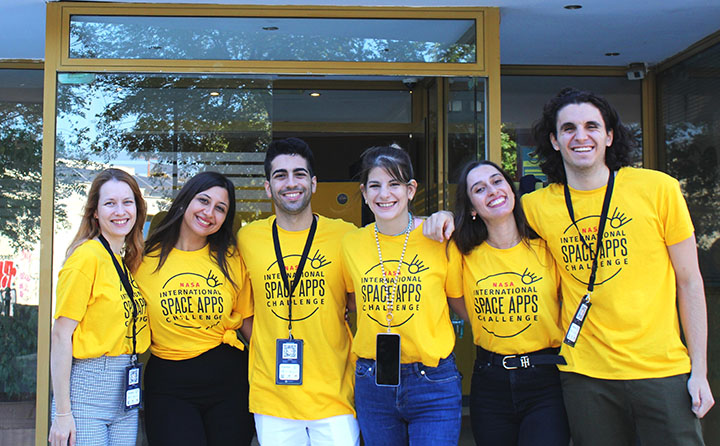
Registration Opens for the 2024 NASA International Space Apps Challenge
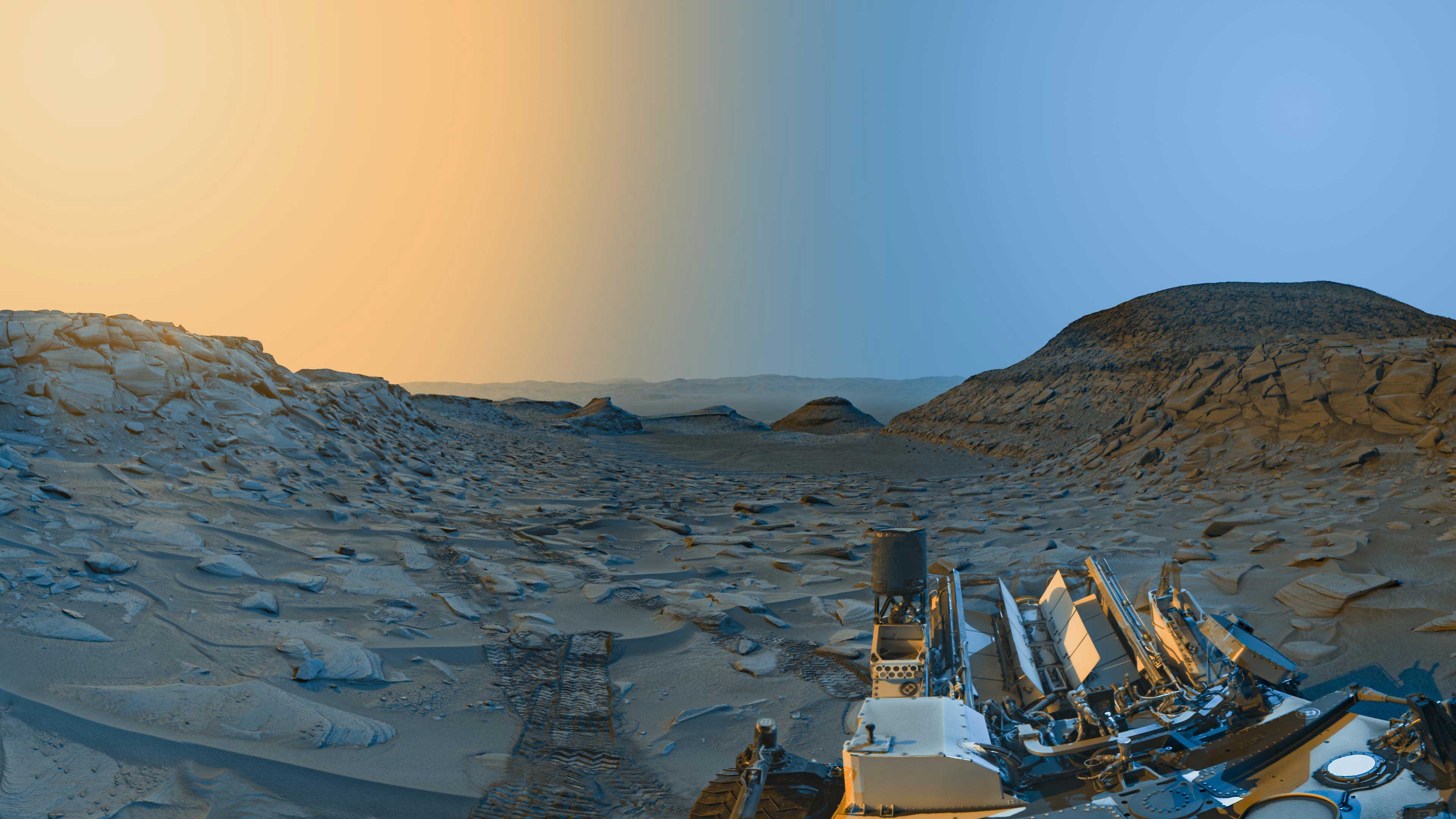
UPDATED: 10 Things for Mars 10
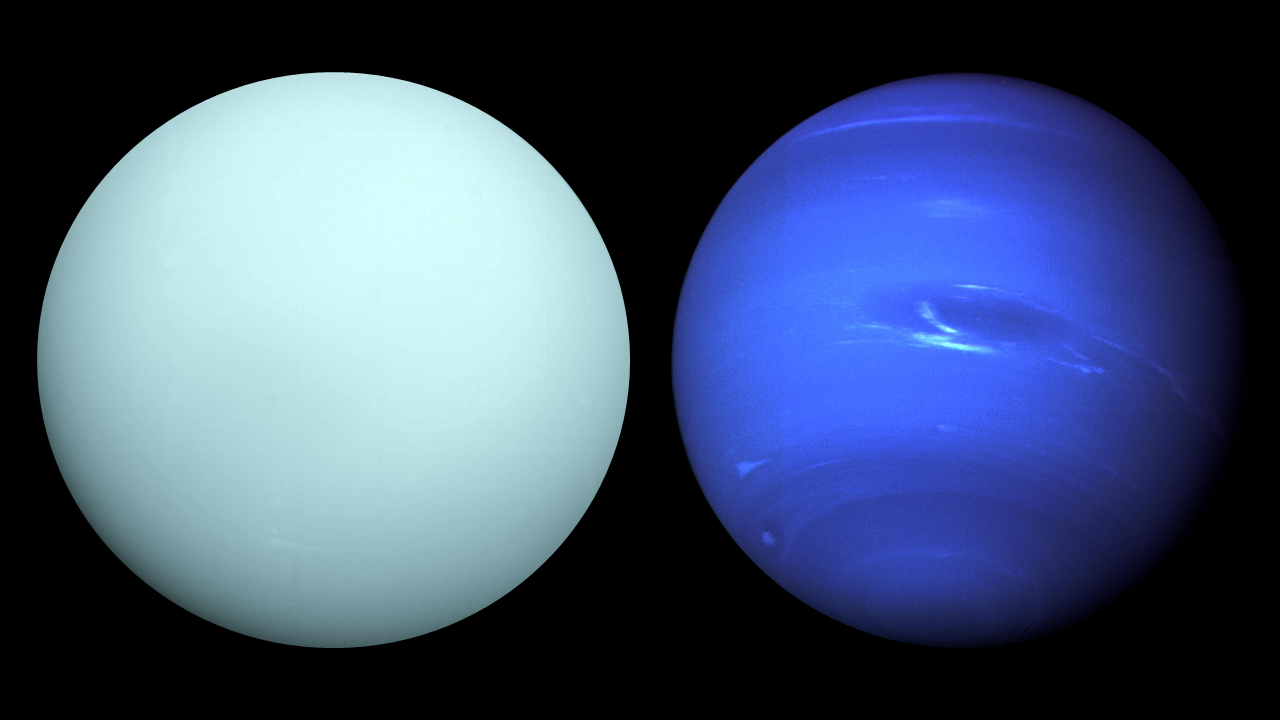
Ice Giant Resources
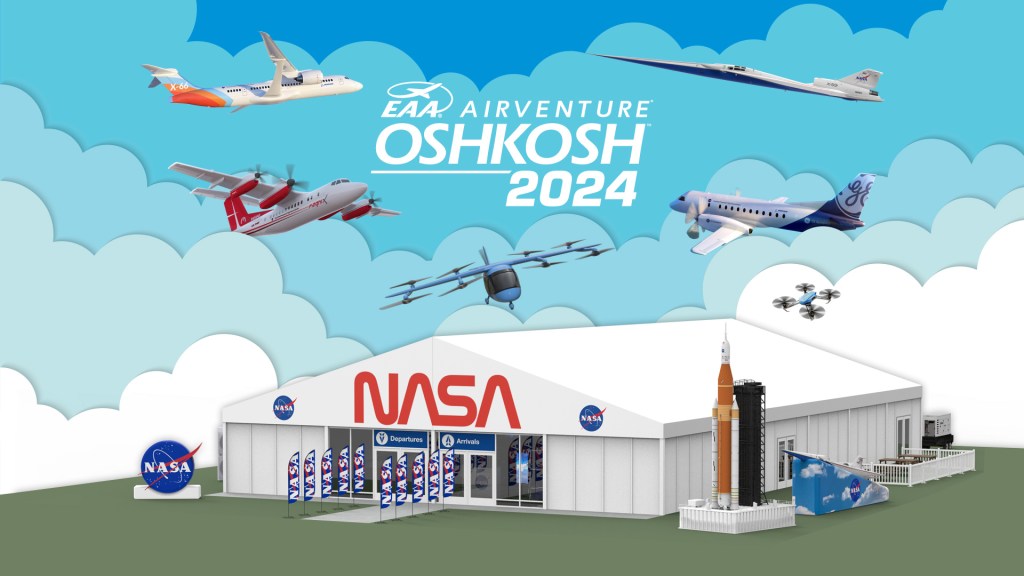
LIVE: NASA is with you from Oshkosh
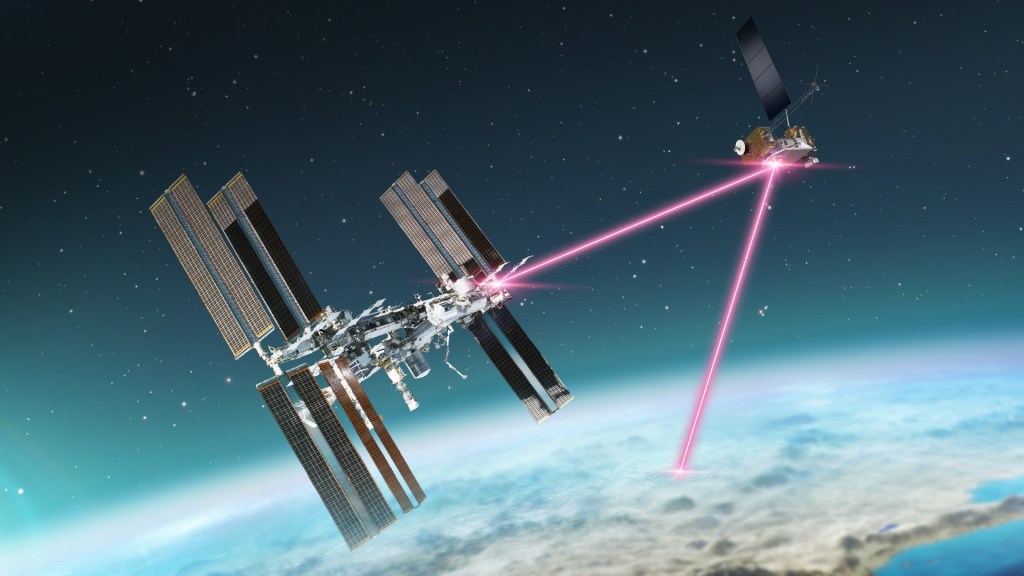
NASA Streams First 4K Video from Aircraft to Space Station, Back
Nasa to host panels, forums, and more at oshkosh 2024.
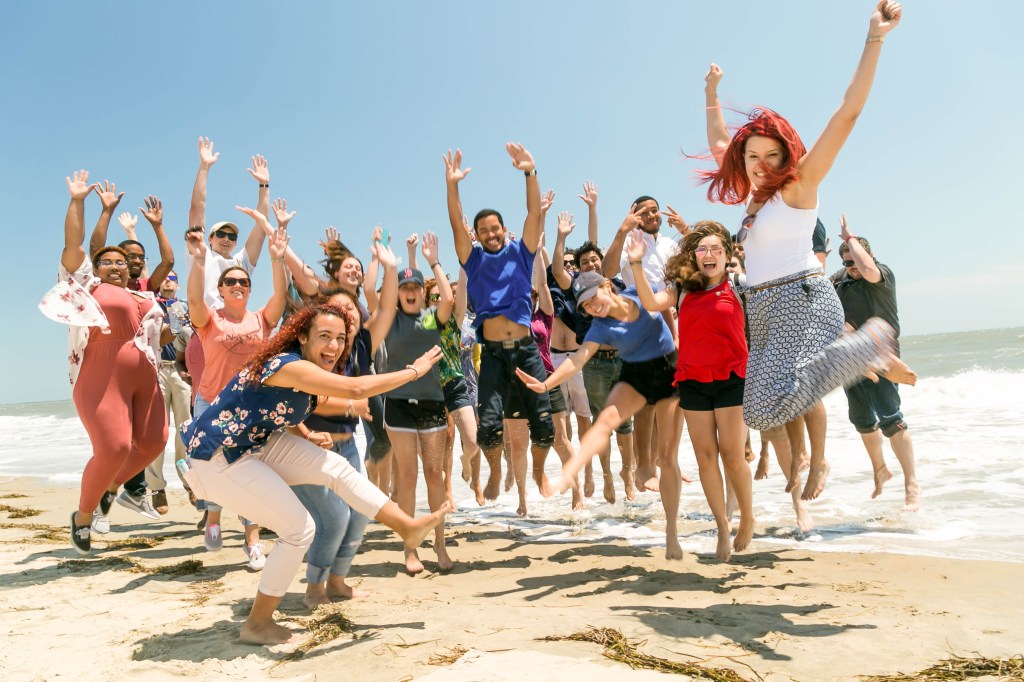
Former Space Communications, Navigation Interns Pioneer NASA’s Future
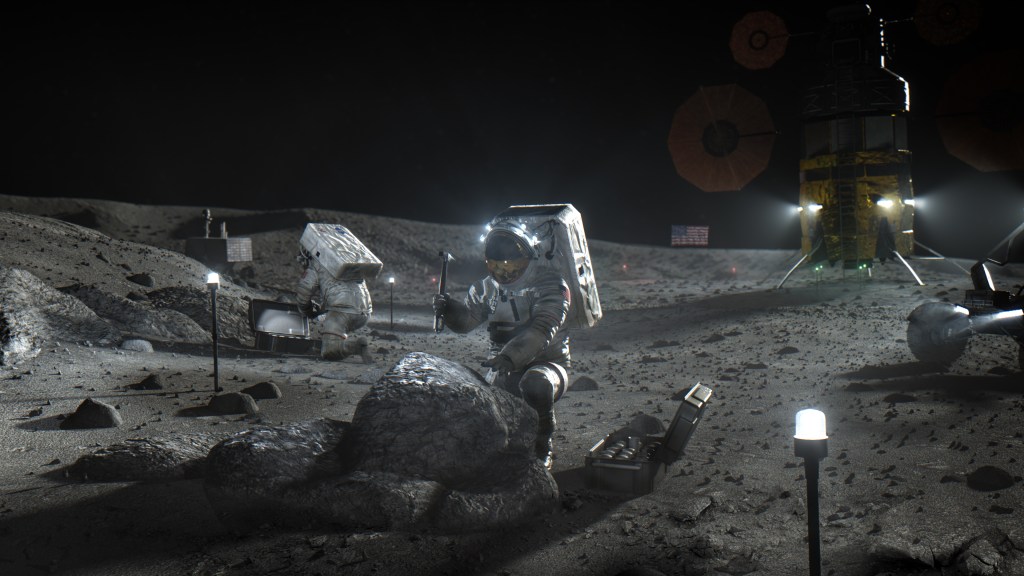
Ground Antenna Trio to Give NASA’s Artemis Campaign ‘LEGS’ to Stand On
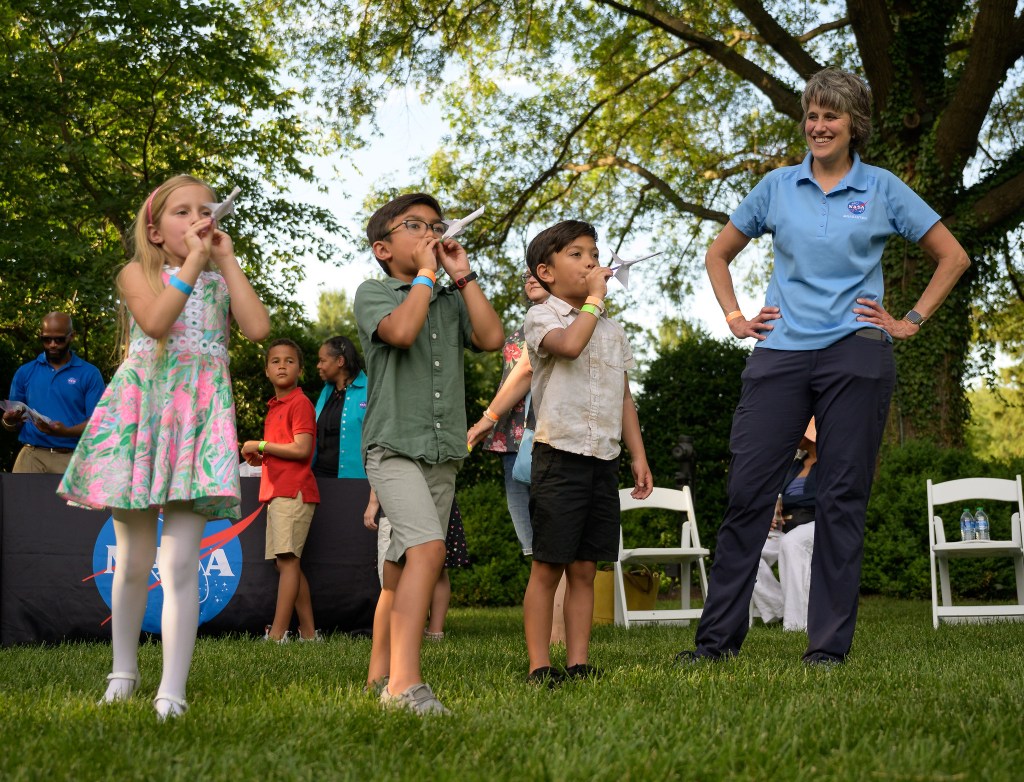
Slow Your Student’s ‘Summer Slide’ and Beat Boredom With NASA STEM

Designing Space Exploration With the Human in Mind
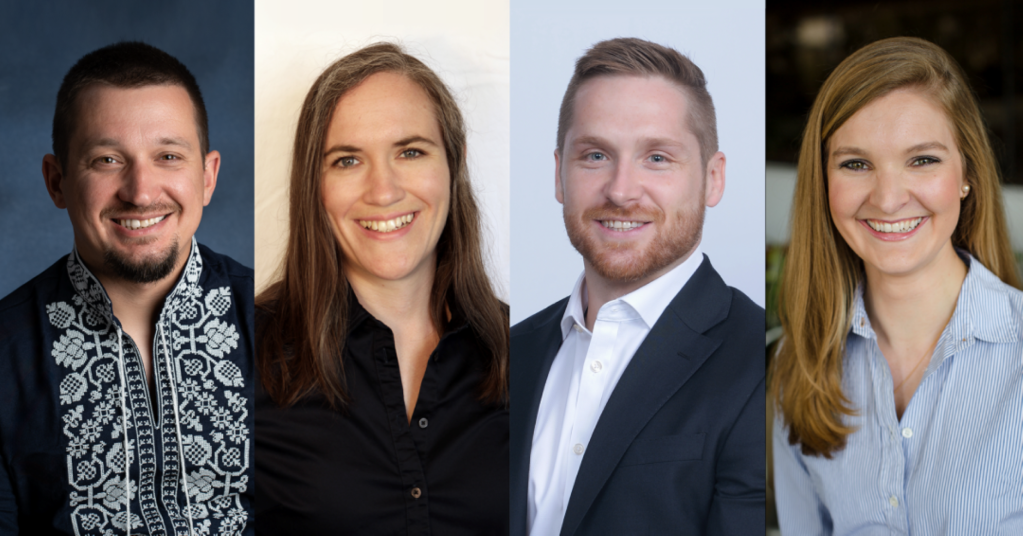
NASA Research Volunteers to Begin Next Simulated Mission to Mars
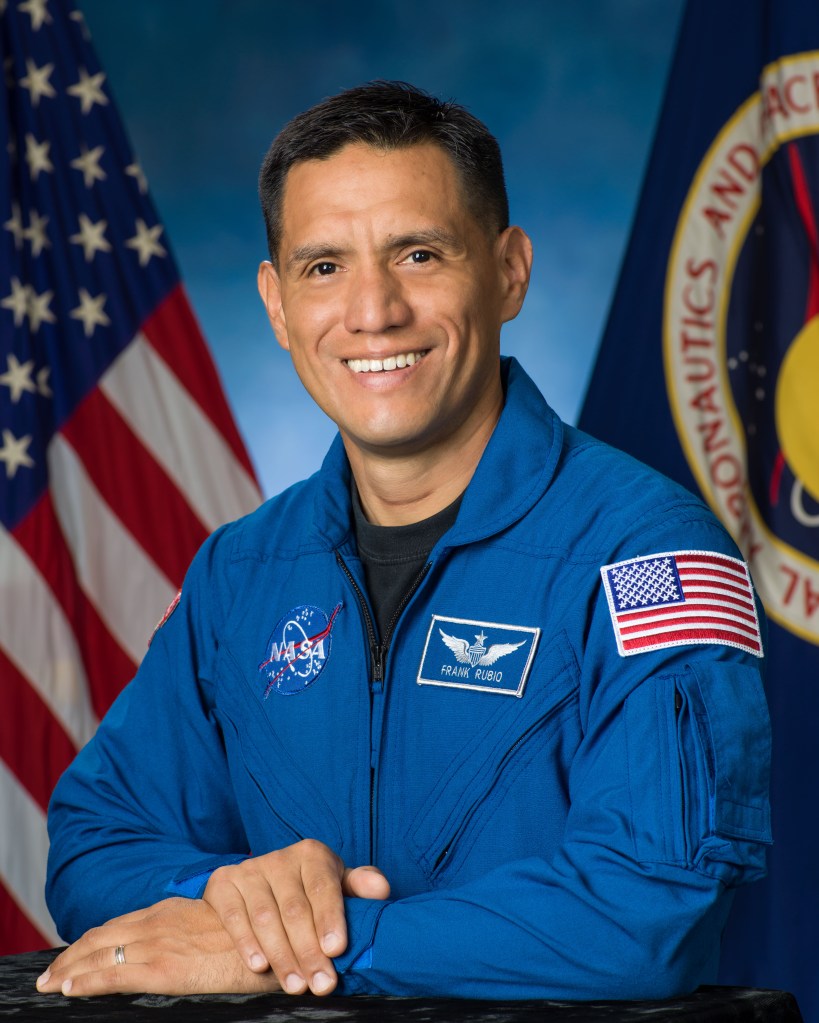
Astronauta de la NASA Frank Rubio
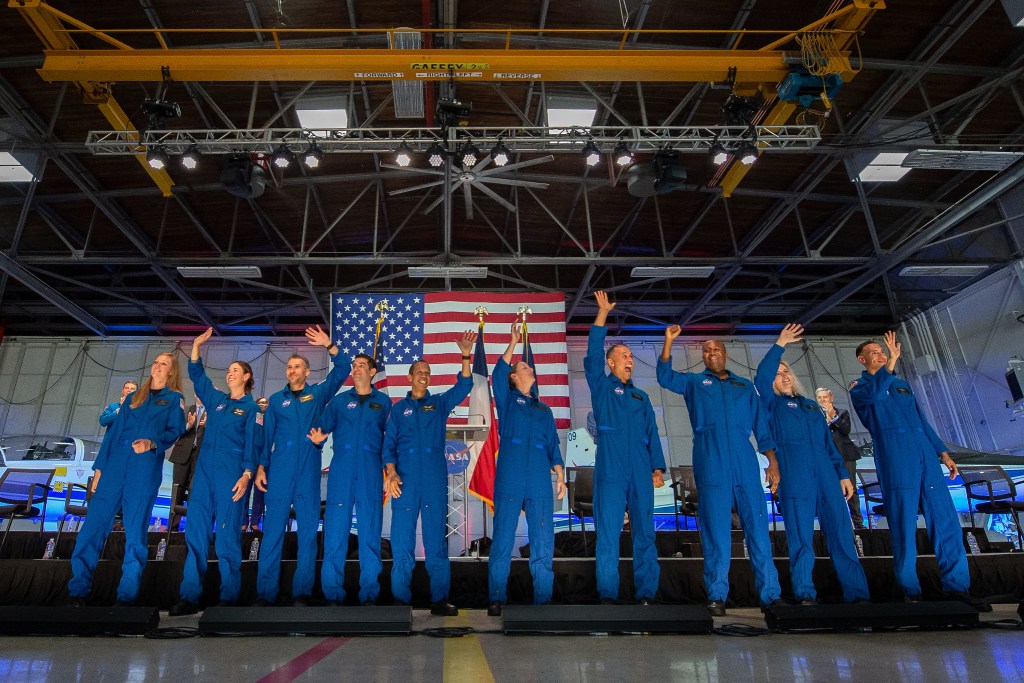
Diez maneras en que los estudiantes pueden prepararse para ser astronautas
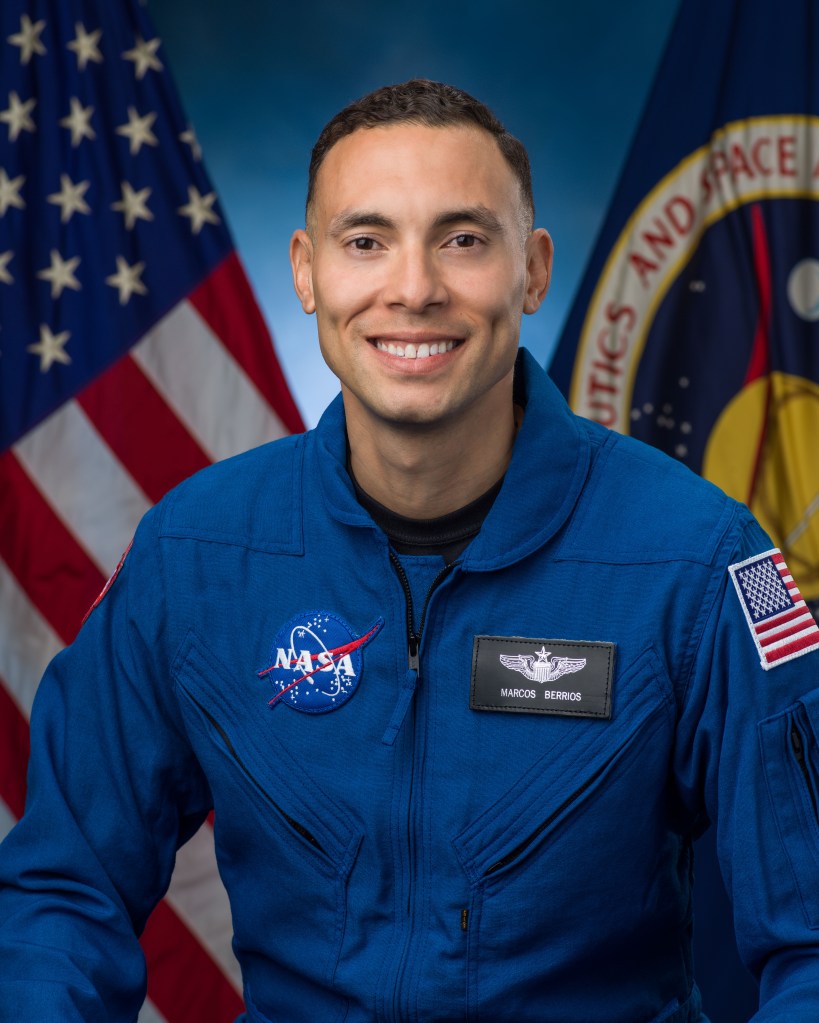
Astronauta de la NASA Marcos Berríos
Nasa’s juno mission captures the colorful and chaotic clouds of jupiter, jet propulsion laboratory.
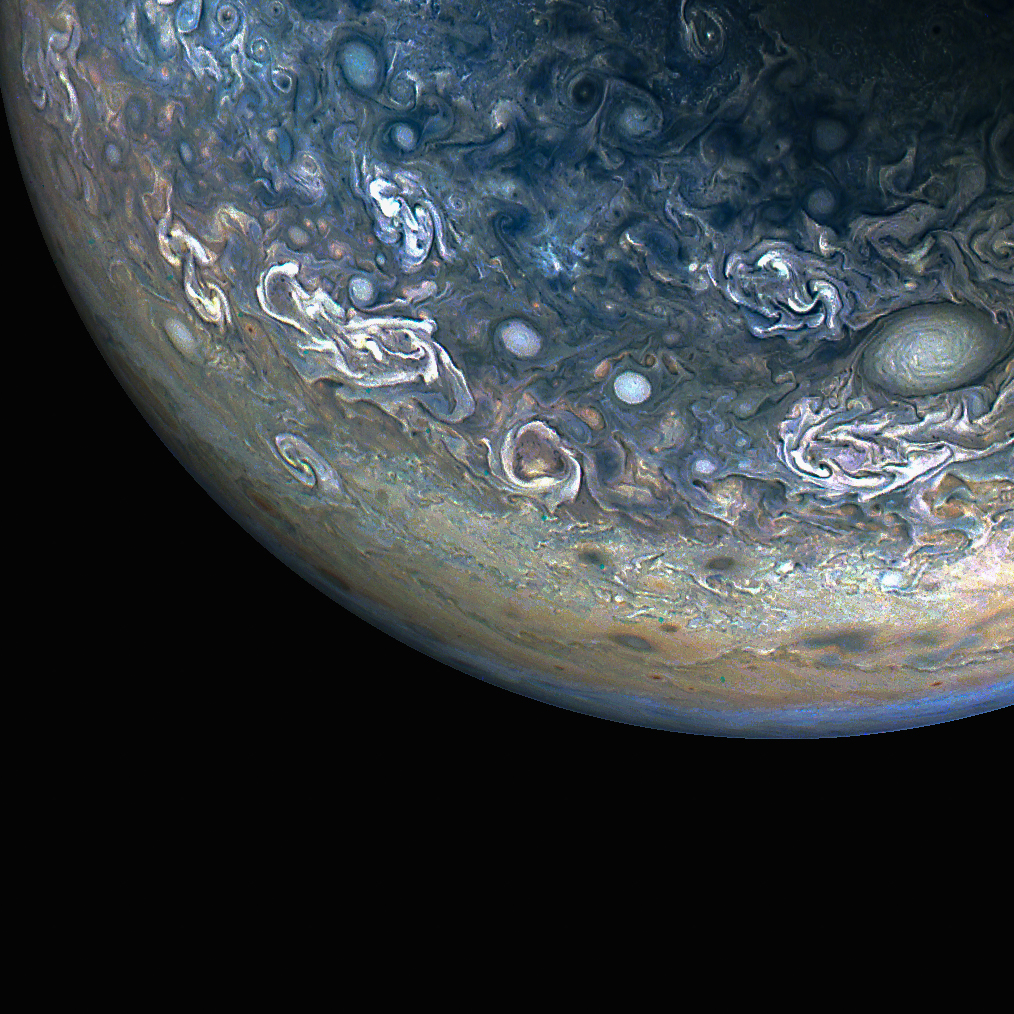
During its 61st close flyby of Jupiter on May 12, 2024, NASA’s Juno spacecraft captured this color-enhanced view of the giant planet’s northern hemisphere. It provides a detailed view of chaotic clouds and cyclonic storms in an area known to scientists as a folded filamentary region. In these regions, the zonal jets that create the familiar banded patterns in Jupiter’s clouds break down, leading to turbulent patterns and cloud structures that rapidly evolve over the course of only a few days.
Citizen scientist Gary Eason made this image using raw data from the JunoCam instrument, applying digital processing techniques to enhance color and clarity.
At the time the raw image was taken, the Juno spacecraft was about 18,000 miles (29,000 kilometers) above Jupiter’s cloud tops, at a latitude of about 68 degrees north of the equator.
JunoCam’s raw images are available for the public to peruse and process into image products at https://missionjuno.swri.edu/junocam/processing . More information about NASA citizen science can be found at https://science.nasa.gov/citizenscience and https://www.nasa.gov/solve/opportunities/citizenscience .
More information about Juno is at https://www.nasa.gov/juno and https://missionjuno.swri.edu . For more about this finding and other science results, see https://www.missionjuno.swri.edu/science-findings .
Suggestions or feedback?
MIT News | Massachusetts Institute of Technology
- Machine learning
- Sustainability
- Black holes
- Classes and programs
Departments
- Aeronautics and Astronautics
- Brain and Cognitive Sciences
- Architecture
- Political Science
- Mechanical Engineering
Centers, Labs, & Programs
- Abdul Latif Jameel Poverty Action Lab (J-PAL)
- Picower Institute for Learning and Memory
- Lincoln Laboratory
- School of Architecture + Planning
- School of Engineering
- School of Humanities, Arts, and Social Sciences
- Sloan School of Management
- School of Science
- MIT Schwarzman College of Computing
Roadmap details how to improve exoplanet exploration using the JWST
Press contact :.
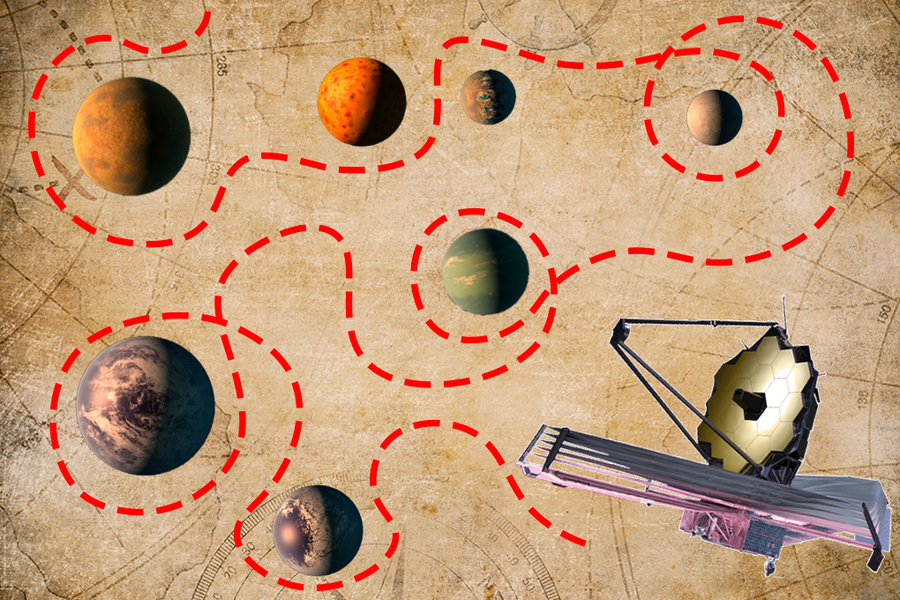
Previous image Next image
The launch of NASA’s James Webb Space Telescope (JWST) in 2021 kicked off an exciting new era for exoplanet research, especially for scientists looking at terrestrial planets orbiting stars other than our sun. But three years into the telescope’s mission, some scientists have run into challenges that have slowed down progress.
In a recent paper published in Nature Astronomy , the TRAPPIST-1 JWST Community Initiative lays out a step-by-step roadmap to overcome the challenges they faced while studying the TRAPPIST-1 system by improving the efficiency of data gathering to benefit the astronomy community at large.
“A whole community of experts came together to tackle these complex cross-disciplinary challenges to design the first multiyear observational strategy to give JWST a fighting chance at identifying habitable worlds over its lifetime,” says Julien de Wit, an associate professor in MIT’s Department of Earth, Atmospheric and Planetary Sciences (EAPS) and one of the lead authors of the paper.
Two-for-one deal
Located 41 light years from Earth, the TRAPPIST-1 system with its seven planets presents a unique opportunity to study a large system with multiple planets of different compositions, similar to our own solar system.
“It's a dream target: You have not one, but maybe three, planets in the habitable zone, so you have a way to actually compare in the same system,” says René Doyon from the Université de Montréal, who co-led the study with de Wit. “There are only a handful of well-characterized temperate rocky planets for which we can hope to detect their atmosphere, and most of them are within the TRAPPIST-1 system.”
Astronomers like de Wit and Doyon study exoplanet atmospheres through a technique called transmission spectroscopy, where they look at the way starlight passes through a planet’s potential atmosphere to see what elements are present. Transmission spectra are collected when the planet passes in front of its host star.
The planets within the TRAPPIST system have short orbital periods. As a result, their transits frequently overlap. Transit observation times are usually allotted in five-hour windows, and when scheduled properly, close to half of these can catch at least two transits. This “two-for-one” saves both time and money while doubling data collection.
Stellar contamination
Stars are not uniform; their surfaces can vary in temperature, creating spots that can be hotter or cooler. Molecules like water vapor can condense in cool spots and interfere with transmission spectra. Stellar information like this can be difficult to disentangle from the planetary signal and give false indications of a planet’s atmospheric composition, creating what’s known as “stellar contamination.” While it has often been ignored, the improved capabilities of the JWST have revealed the challenges stellar contamination introduces when studying planetary atmospheres.
EAPS research scientist Ben Rackham ran into these challenges when they derailed his initial PhD research on small exoplanets using the Magellan Telescopes in Chile. He’s now seeing the same problem he first encountered as a graduate student repeating itself with the new JWST data.
“As we predicted from that earlier work with data from ground-based telescopes, the very first spectral signatures we're getting with JWST don't really make any sense in terms of a planetary interpretation,” he says. “The features are not what we would expect to see, and they change from transit to transit.”
Rackham and David Berardo, a postdoc in EAPS, have been working with de Wit on ways to correct for stellar contamination using two different methods: improving models of stellar spectra and using direct observations to derive corrections .
“By observing a star as it rotates, we can use the sensitivity of JWST to get a clearer picture of what its surface looks like, allowing for a more accurate measuring of the atmosphere of planets that transit it,” says Berardo. This, combined with studying back-to-back transits as proposed in the roadmap, collects useful data on the star that can be used to filter out stellar contamination from both future studies and past ones.
Beyond TRAPPIST-1
The current roadmap was born from the efforts of the TRAPPIST JWST Community Initiative to bring together separate programs focused on individual planets, which prevented them from leveraging the optimal transit observation windows.
“We understood early on that this effort would 'take a village' to avoid the efficiency traps of small observation programs , ” says de Wit. “Our hope now is that a large-scale community effort guided by the roadmap can be initiated to yield deliverables at a timely pace.” De Wit hopes that it could result in identifying habitable, or inhabitable, worlds around TRAPPIST-1 within a decade.
Both de Wit and Doyon believe that the TRAPPIST-1 system is the best place for conducting fundamental research on exoplanet atmospheres that will extend to studies in other systems. Doyon thinks that “the TRAPPIST-1 system will be useful not only for TRAPPIST-1 itself, but also to learn how to do very precise correction of stellar activity which will be beneficial to many other transmission spectroscopy programs also affected by stellar activity.”
“We have within reach fundamental and transforming answers with a clear roadmap to them,” says de Wit. “We just need to follow it diligently.”
Share this news article on:
Related links.
- Julien de Wit
- MIT Kavli Institute for Astrophysics and Space Research
- Department of Earth, Atmospheric and Planetary Sciences
Related Topics
- Space, astronomy and planetary science
- Collaboration
Related Articles
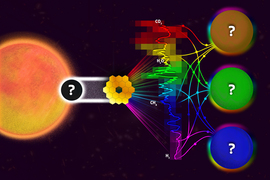
Study: Astronomers risk misinterpreting planetary signals in JWST data
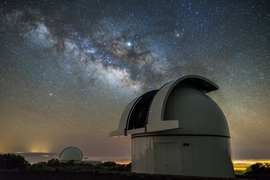
3Q: Julien de Wit on searching for red worlds in the northern skies
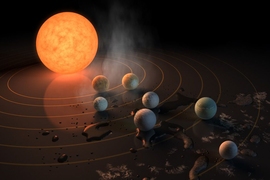
3Q: Julien de Wit on the discovery of seven temperate, nearby worlds
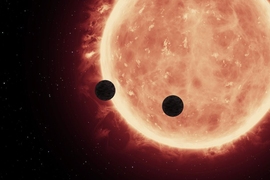
First atmospheric study of Earth-sized exoplanets reveals rocky worlds
Previous item Next item
More MIT News
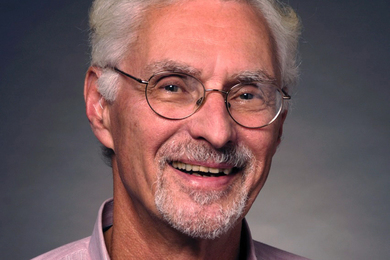

Professor Emeritus Ralph Gakenheimer, mobility planner and champion of international development, dies at 89
Read full story →
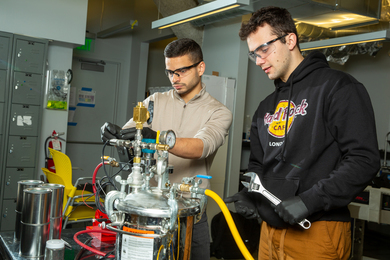
A recipe for zero-emissions fuel: Soda cans, seawater, and caffeine
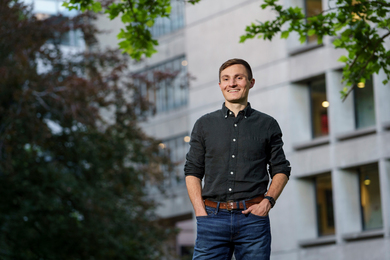
Balancing economic development with natural resources protection
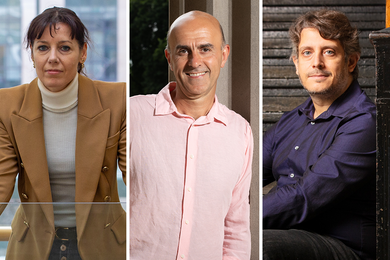
Three MIT professors named 2024 Vannevar Bush Fellows
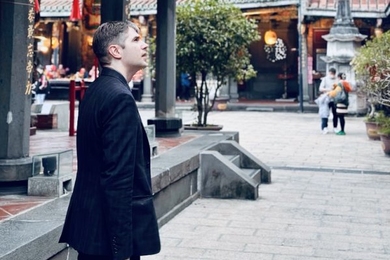
Q&A: “As long as you have a future, you can still change it”
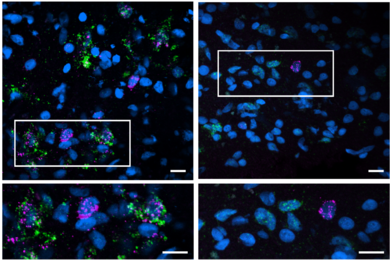
Study across multiple brain regions discerns Alzheimer’s vulnerability and resilience factors
- More news on MIT News homepage →
Massachusetts Institute of Technology 77 Massachusetts Avenue, Cambridge, MA, USA
- Map (opens in new window)
- Events (opens in new window)
- People (opens in new window)
- Careers (opens in new window)
- Accessibility
- Social Media Hub
- MIT on Facebook
- MIT on YouTube
- MIT on Instagram
You are using an outdated browser. Please upgrade your browser to improve your experience.
share this!
July 24, 2024
This article has been reviewed according to Science X's editorial process and policies . Editors have highlighted the following attributes while ensuring the content's credibility:
fact-checked
peer-reviewed publication
trusted source
Roadmap details how to improve exoplanet exploration using the James Webb Space Telescope
by Paige Colley, Massachusetts Institute of Technology
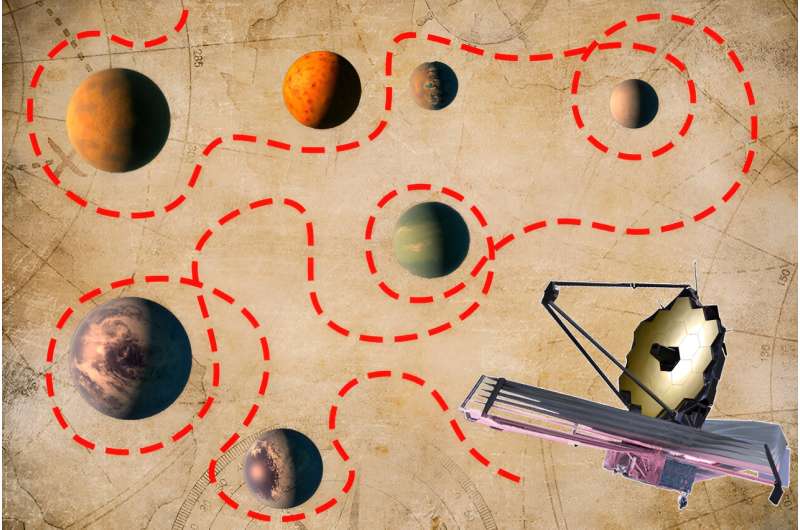
The launch of NASA's James Webb Space Telescope (JWST) in 2021 kicked off an exciting new era for exoplanet research, especially for scientists looking at terrestrial planets orbiting stars other than our sun. But three years into the telescope's mission, some scientists have run into challenges that have slowed down progress.
In a recent paper published in Nature Astronomy , the TRAPPIST-1 JWST Community Initiative lays out a step-by-step roadmap to overcome the challenges they faced while studying the TRAPPIST-1 system by improving the efficiency of data gathering to benefit the astronomy community at large.
"A whole community of experts came together to tackle these complex cross-disciplinary challenges to design the first multiyear observational strategy to give JWST a fighting chance at identifying habitable worlds over its lifetime," says Julien de Wit, an associate professor in MIT's Department of Earth, Atmospheric and Planetary Sciences (EAPS) and one of the lead authors of the paper.
Two-for-one deal
Located 41 light years from Earth, the TRAPPIST-1 system, with its seven planets, presents a unique opportunity to study a large system with multiple planets of different compositions, similar to our own solar system.
"It's a dream target: You have not one, but maybe three planets in the habitable zone , so you have a way to actually compare in the same system," says René Doyon from the Université de Montréal, who co-led the study with de Wit. "There are only a handful of well-characterized temperate rocky planets for which we can hope to detect their atmosphere, and most of them are within the TRAPPIST-1 system."
Astronomers like de Wit and Doyon study exoplanet atmospheres through a technique called transmission spectroscopy, where they look at the way starlight passes through a planet's potential atmosphere to see what elements are present. Transmission spectra are collected when the planet passes in front of its host star.
The planets within the TRAPPIST system have short orbital periods. As a result, their transits frequently overlap. Transit observation times are usually allotted in five-hour windows, and when scheduled properly, close to half of these can catch at least two transits. This "two-for-one" saves both time and money while doubling data collection.
Stellar contamination
Stars are not uniform; their surfaces can vary in temperature, creating spots that can be hotter or cooler. Molecules like water vapor can condense in cool spots and interfere with transmission spectra. Stellar information like this can be difficult to disentangle from the planetary signal and give false indications of a planet's atmospheric composition, creating what's known as "stellar contamination." While it has often been ignored, the improved capabilities of the JWST have revealed the challenges stellar contamination introduces when studying planetary atmospheres.
EAPS research scientist Ben Rackham ran into these challenges when they derailed his initial Ph.D. research on small exoplanets using the Magellan Telescopes in Chile. He's now seeing the same problem he first encountered as a graduate student repeating itself with the new JWST data.
"As we predicted from that earlier work with data from ground-based telescopes , the very first spectral signatures we're getting with JWST don't really make any sense in terms of a planetary interpretation," he says. "The features are not what we would expect to see, and they change from transit to transit."
Rackham and David Berardo, a postdoc in EAPS, have been working with de Wit on ways to correct for stellar contamination using two different methods: improving models of stellar spectra and using direct observations to derive corrections .
"By observing a star as it rotates, we can use the sensitivity of JWST to get a clearer picture of what its surface looks like, allowing for a more accurate measuring of the atmosphere of planets that transit it," says Berardo. This, combined with studying back-to-back transits as proposed in the roadmap, collects useful data on the star that can be used to filter out stellar contamination from both future studies and past ones.
Beyond TRAPPIST-1
The current roadmap was born from the efforts of the TRAPPIST JWST Community Initiative to bring together separate programs focused on individual planets, which prevented them from leveraging the optimal transit observation windows.
"We understood early on that this effort would 'take a village' to avoid the efficiency traps of small observation programs," says de Wit. "Our hope now is that a large-scale community effort guided by the roadmap can be initiated to yield deliverables at a timely pace."
De Wit hopes that it could result in identifying habitable, or inhabitable, worlds around TRAPPIST-1 within a decade.
Both de Wit and Doyon believe that the TRAPPIST-1 system is the best place for conducting fundamental research on exoplanet atmospheres that will extend to studies in other systems. Doyon thinks that "the TRAPPIST-1 system will be useful not only for TRAPPIST-1 itself, but also to learn how to do very precise correction of stellar activity which will be beneficial to many other transmission spectroscopy programs also affected by stellar activity."
"We have within reach fundamental and transforming answers with a clear roadmap to them," says de Wit. "We just need to follow it diligently."
Journal information: Nature Astronomy
Provided by Massachusetts Institute of Technology
This story is republished courtesy of MIT News ( web.mit.edu/newsoffice/ ), a popular site that covers news about MIT research, innovation and teaching.
Explore further
Feedback to editors

Perseverance rover discovers rock with potential signs of ancient life
13 minutes ago

Fermi Telescope finds new feature in brightest gamma-ray burst yet seen
37 minutes ago

Confined water gets electric: Study reveals dielectric response of water in nanopores

Underground CO₂ storage: Researchers measure carbon mineralization at unprecedented small scale

Climate change will bring more turbulence to flights in the Northern Hemisphere, analysis finds

Liquid metals offer potential for greener chemical processes, researchers say

Increasingly erratic rainfall patterns over the past century are likely due to human-induced climate change, study shows

Somersaulting spin qubits for universal quantum logic could enhance control in larger arrays

Raindrops grow with turbulence in clouds: New findings could improve weather and climate models
2 hours ago

NASA says no return date yet for astronauts and troubled Boeing capsule at space station
3 hours ago
Relevant PhysicsForums posts
Solar activity and space weather update thread.
Jul 24, 2024
Some photos of Antares and Sigma Sagittarii (Nunki)
Jul 19, 2024
Shell structure topological defects as substitute for dark matter
Star like object travels in a zigzagging pattern relative to other stars.
Jul 18, 2024
The James Webb Space Telescope
Jul 13, 2024
Our Beautiful Universe - Photos and Videos
More from Astronomy and Astrophysics
Related Stories

James Webb Space Telescope's first spectrum of a TRAPPIST-1 planet
Sep 25, 2023

TRAPPIST-1 outer planets likely have water, research suggests
May 15, 2024

TRAPPIST-1c isn't the exo-Venus we were hoping for, but don't blame the star
Jan 2, 2024

Atmospheres in the TRAPPIST-1 system should be long gone
Feb 5, 2024

Looking for atmospheres in the ultimate quest for extraterrestrial life
Jun 26, 2024

Astronomers find surprising ice world in the habitable zone with JWST data
Jul 8, 2024
Recommended for you

Geoscientists narrow timing of enormous 'magmatic event' on the moon more precisely
4 hours ago

Carbon oxides on Uranus' moon Ariel hint at hidden ocean, Webb telescope reveals
5 hours ago

Webb images nearest super-Jupiter, opening a new window to exoplanet research

Chinese lunar probe finds water in moon samples
Jul 23, 2024

Effects of stellar magnetism could expand criteria for exoplanet habitability
Jul 22, 2024

New dawn for space storm alerts could help shield Earth's tech
Let us know if there is a problem with our content.
Use this form if you have come across a typo, inaccuracy or would like to send an edit request for the content on this page. For general inquiries, please use our contact form . For general feedback, use the public comments section below (please adhere to guidelines ).
Please select the most appropriate category to facilitate processing of your request
Thank you for taking time to provide your feedback to the editors.
Your feedback is important to us. However, we do not guarantee individual replies due to the high volume of messages.
E-mail the story
Your email address is used only to let the recipient know who sent the email. Neither your address nor the recipient's address will be used for any other purpose. The information you enter will appear in your e-mail message and is not retained by Phys.org in any form.
Newsletter sign up
Get weekly and/or daily updates delivered to your inbox. You can unsubscribe at any time and we'll never share your details to third parties.
More information Privacy policy
Donate and enjoy an ad-free experience
We keep our content available to everyone. Consider supporting Science X's mission by getting a premium account.
E-mail newsletter
Thank you for visiting nature.com. You are using a browser version with limited support for CSS. To obtain the best experience, we recommend you use a more up to date browser (or turn off compatibility mode in Internet Explorer). In the meantime, to ensure continued support, we are displaying the site without styles and JavaScript.
- View all journals
- Explore content
- About the journal
- Publish with us
- Sign up for alerts
- Open access
- Published: 17 July 2024
A hot-Jupiter progenitor on a super-eccentric retrograde orbit
- Arvind F. Gupta ORCID: orcid.org/0000-0002-5463-9980 1 , 2 , 3 ,
- Sarah C. Millholland ORCID: orcid.org/0000-0003-3130-2282 4 , 5 ,
- Haedam Im ORCID: orcid.org/0009-0006-0871-1618 4 , 5 ,
- Jiayin Dong ORCID: orcid.org/0000-0002-3610-6953 6 ,
- Jonathan M. Jackson ORCID: orcid.org/0000-0002-0323-4828 7 ,
- Ilaria Carleo ORCID: orcid.org/0000-0002-0810-3747 8 , 9 ,
- Jessica Libby-Roberts 2 , 3 ,
- Megan Delamer 2 , 3 ,
- Mark R. Giovinazzi 10 ,
- Andrea S. J. Lin 2 , 3 ,
- Shubham Kanodia 11 ,
- Xian-Yu Wang 12 ,
- Keivan Stassun ORCID: orcid.org/0000-0002-3481-9052 13 ,
- Thomas Masseron 8 , 9 ,
- Diana Dragomir ORCID: orcid.org/0000-0003-2313-467X 14 ,
- Suvrath Mahadevan ORCID: orcid.org/0000-0001-9596-7983 2 , 3 ,
- Jason Wright 2 , 3 , 15 ,
- Jaime A. Alvarado-Montes ORCID: orcid.org/0000-0003-0353-9741 16 , 17 ,
- Chad Bender ORCID: orcid.org/0000-0003-4384-7220 18 ,
- Cullen H. Blake 10 ,
- Douglas Caldwell 19 ,
- Caleb I. Cañas 20 ,
- William D. Cochran ORCID: orcid.org/0000-0001-9662-3496 21 , 22 ,
- Paul Dalba 23 ,
- Mark E. Everett 1 ,
- Pipa Fernandez 1 ,
- Eli Golub 1 ,
- Bruno Guillet ORCID: orcid.org/0000-0003-4091-0247 na1 ,
- Samuel Halverson 24 ,
- Leslie Hebb 25 , 26 ,
- Jesus Higuera 1 ,
- Chelsea X. Huang 27 ,
- Jessica Klusmeyer ORCID: orcid.org/0000-0003-3906-9518 1 ,
- Rachel Knight na1 ,
- Liouba Leroux ORCID: orcid.org/0000-0003-3046-9187 na1 ,
- Sarah E. Logsdon ORCID: orcid.org/0000-0002-9632-9382 1 ,
- Margaret Loose ORCID: orcid.org/0000-0002-5215-4779 na1 ,
- Michael W. McElwain ORCID: orcid.org/0000-0003-0241-8956 20 ,
- Andrew Monson 18 ,
- Joe P. Ninan 28 ,
- Grzegorz Nowak ORCID: orcid.org/0000-0002-7031-7754 8 , 9 , 29 ,
- Enric Palle ORCID: orcid.org/0000-0003-0987-1593 8 , 9 ,
- Yatrik Patel 1 ,
- Joshua Pepper ORCID: orcid.org/0000-0002-3827-8417 30 ,
- Michael Primm ORCID: orcid.org/0000-0003-3462-7533 na1 ,
- Jayadev Rajagopal 1 ,
- Paul Robertson 31 ,
- Arpita Roy ORCID: orcid.org/0000-0001-8127-5775 32 ,
- Donald P. Schneider 2 , 3 ,
- Christian Schwab 16 , 17 ,
- Heidi Schweiker 1 ,
- Lauren Sgro 19 ,
- Masao Shimizu ( 清水正雄 ) na1 ,
- Georges Simard na1 ,
- Guðmundur Stefánsson 33 , 34 ,
- Daniel J. Stevens ORCID: orcid.org/0000-0002-5951-8328 35 ,
- Steven Villanueva 20 ,
- John Wisniewski 36 ,
- Stefan Will ORCID: orcid.org/0000-0003-0404-6279 na1 &
- Carl Ziegler 37
Nature ( 2024 ) Cite this article
4329 Accesses
559 Altmetric
Metrics details
- Giant planets
Giant exoplanets orbiting close to their host stars are unlikely to have formed in their present configurations 1 . These ‘hot Jupiter’ planets are instead thought to have migrated inward from beyond the ice line and several viable migration channels have been proposed, including eccentricity excitation through angular-momentum exchange with a third body followed by tidally driven orbital circularization 2 , 3 . The discovery of the extremely eccentric ( e = 0.93) giant exoplanet HD 80606 b (ref. 4 ) provided observational evidence that hot Jupiters may have formed through this high-eccentricity tidal-migration pathway 5 . However, no similar hot-Jupiter progenitors have been found and simulations predict that one factor affecting the efficacy of this mechanism is exoplanet mass, as low-mass planets are more likely to be tidally disrupted during periastron passage 6 , 7 , 8 . Here we present spectroscopic and photometric observations of TIC 241249530 b, a high-mass, transiting warm Jupiter with an extreme orbital eccentricity of e = 0.94. The orbit of TIC 241249530 b is consistent with a history of eccentricity oscillations and a future tidal circularization trajectory. Our analysis of the mass and eccentricity distributions of the transiting-warm-Jupiter population further reveals a correlation between high mass and high eccentricity.
Similar content being viewed by others
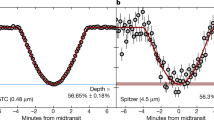
A giant planet candidate transiting a white dwarf
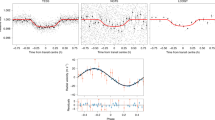
An ultrahot Neptune in the Neptune desert
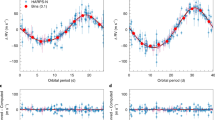
Rapid contraction of giant planets orbiting the 20-million-year-old star V1298 Tau
The Transiting Exoplanet Survey Satellite (TESS) 9 monitored the apparent brightness of the star TIC 241249530 for 28 days during the second year of its primary mission. These data reveal a transit-like approximately 0.8% dip in brightness on 12 January 2020, the shape and depth of which were consistent with a Jupiter-sized planet passing in front of the star (Fig. 1a ). To find out the nature and origin of this signal, we conducted a series of ground-based observations of TIC 241249530. We first used high-spatial-resolution speckle imaging data from NESSI 10 to rule out the presence of contaminating sources and confirm that the signal was not associated with a background eclipsing-binary in the TESS aperture. We then began radial velocity (RV) observations with the NEID spectrograph 11 , which revealed that the TESS transit was probably induced by a giant exoplanet on a highly eccentric ( e = 0.94), long-period ( P = 167 days) orbit. These measurements were consistent with the absence of a transit detection when TESS re-observed this star for 27 days from December 2022 to January 2023. Further NEID measurements, supplemented by observations with the HPF 12 and HARPS-N 13 spectrographs, were strategically scheduled to be taken when the planet was predicted to be approaching periastron and thus inducing large stellar RV variations. We attempted to detect a second transit using the global Unistellar telescope network 14 in March 2023, but these efforts were unsuccessful as the ephemeris was not yet well constrained. However, RV data collected during the periastron window enabled us to more precisely predict the subsequent transit window. We captured the first half of this transit using the engineered diffuser 15 on the ARCTIC imager 16 on 30 August 2023 (Fig. 1b ). We refined the orbit using the ARCTIC data together with further NEID observations, including several concurrent with this transit and the subsequent one on 12 February 2024. Our ensemble of photometric and RV measurements is best explained by a massive exoplanet on a long-period, eccentric orbit.
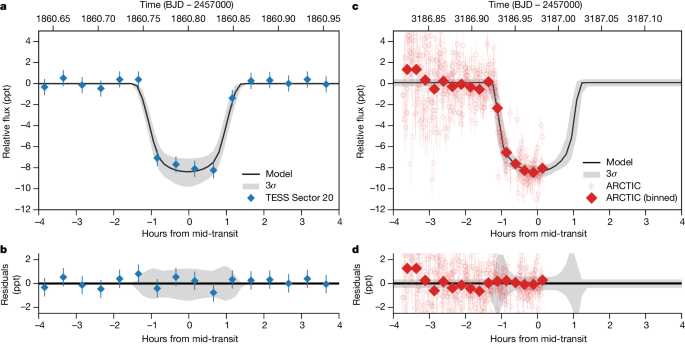
a , TESS photometric measurements (blue), shown at their native 30-min cadence, and the best-fit transit model (black curve) and 3 σ confidence region (grey). b , Residuals to the best-fit model for the TESS transit detection. c , Diffuser-assisted ARCTIC photometric measurements (red) and the best-fit transit model (black curve) and 3 σ confidence region (grey). We show both the raw 30-s cadence and binned 15-min cadence measurements, along with the residuals to the fitted transit signal. d , Residuals to the best-fit model for the ARCTIC transit detection. All brightnesses are given in parts per thousand (ppt). Error bars on individual data points indicate the 1 σ measurement uncertainties.
Source Data
To characterize the host star TIC 241249530, we separately analysed the NEID and HARPS-N spectra using synthetic spectral fitting techniques and we then fit the spectral energy distribution (SED; see Methods ). TIC 241249530 is a main-sequence star that is slightly hotter, larger and more massive than the Sun; the derived parameters, listed in Extended Data Table 1 , suggest that the star is 3.2 ± 0.5 Gyr old. The star also has a low-mass binary stellar companion, TIC 241249532, at a projected separation of 4.930 ± 0.104″, or 1,664 ± 11 au .
We jointly fit the NEID, HPF, HARPS-N, TESS and ARCTIC measurements, accounting for perturbations to the in-transit RV signal owing to the Rossiter–McLaughlin effect. The transit and RV fits are shown in Figs. 1 and 2 , respectively, and the best-fit parameters are given in Table 1 . TIC 241249530 b is an exoplanet that is \({4.98}_{-0.18}^{+0.16}\) times as massive as Jupiter and it is on a \({165.77190}_{-0.00028}^{+0.00027}{\rm{-day}}\) orbit around its host star, with an eccentricity of \({0.9412}_{-0.0009}^{+0.0009}\) . Our fit to the Rossiter–McLaughlin signal (Fig. 2b ) shows that the exoplanet is orbiting in the opposite direction to the projected stellar spin \((\lambda ={{163.5}_{-7.7}^{+9.4}}^{\circ })\) and is retrograde to 99.5% confidence. Few exoplanets have orbits as extreme as this; this orbit is more eccentric than that of any other transiting exoplanet, and only a handful of known planets have similarly large projected spin–orbit misalignments 17 .

a , RV measurements from NEID (blue), HPF (black), HARPS-N (yellow) and best-fit orbit model (black curve). b , Residuals to the RV orbit fit. c , Best-fit Rossiter–McLaughlin model (solid curve) and 1 σ confidence region (grey), with the signal for aligned orbit shown for comparison (dashed curve). d , Residuals to the Rossiter–McLaughlin model fit. The dashed red box in a highlights the in-transit RV measurements, which are shown in b . Error bars on individual data points indicate the 1 σ measurement uncertainties.
The planet that most closely resembles TIC 241249530 b is HD 80606 b (ref. 4 ), which has a mass 4.1 times that of Jupiter and is also on a misaligned orbit with a period of 111 days and an eccentricity of 0.93. HD 80606 b is an archetypal example of an exoplanet destined to become a hot Jupiter with an eventual orbital period of less than 10 days. The eccentric orbit carries the planet close enough to its host star at periastron that tides raised on the planet and star will sap energy from the orbit, causing it to gradually shrink and circularize. Also, simulations 3 , 5 show that the present orbit of HD 80606 b is consistent with a history of von Zeipel–Lidov–Kozai (vZLK) eccentricity oscillations 18 , 19 , 20 driven by angular momentum exchange with HD 80607, the stellar companion to the host star. Our own simulations of the dynamical history and trajectory of TIC 241249530 b (see Methods ) show that the orbit is consistent with this same type of perturber-coupled, high-eccentricity tidal migration. Eccentricity oscillations would have continued until the most recent few hundred million years, at which point general relativistic precession overtook the torque exerted by the companion, locking the exoplanet on an eccentric orbit that is now gradually circularizing. The architectures of the HD 80606 and TIC 241249530 systems lend support to this process as a plausible hot-Jupiter-formation mechanism. However, although other giant exoplanets on tidal migration tracks have been discovered 21 , including two that probably have vZLK-driven dynamical histories 22 , 23 , no previous examples have eccentricities >0.9 and none have formation scenarios as clear as that of HD 80606 b. The observed occurrence rate of super-eccentric progenitors to hot Jupiters 24 , 25 falls well short of predictions from simulations 26 , suggesting that giant-planet migration is dominated by other channels. With the discovery of TIC 241249530 b, a second super-eccentric exoplanet in a hierarchical triple system has been added to the sparse sample, providing a new lens through which we can explore the formation of these planets.
Not only do the TIC 241249530 b and HD 80606 b systems share similar orbital architectures but these exoplanets also have similar masses. The masses and eccentricities of all transiting warm Jupiters, which we define as giant planets with intermediate periods (10 days < P < 365 days), are shown in Fig. 3b . These two planets, which are the only members of the sample with super-eccentric orbits ( e > 0.9), are also among the most massive. A correlation between exoplanet mass and eccentricity has been identified in previous works 27 , 28 , 29 , 30 , each of which found that higher-mass planets are more likely to have larger orbital eccentricities. We find that our narrower sample of transiting giant planets conforms to this known trend (see Methods ); the eccentricity distributions of high-mass ( M p > 2 M J ) and low-mass (0.3 M J ≤ M p ≤ 2 M J ) members of this population are statistically distinct (Fig. 3a ). Although lower-mass planets are more likely to be found on low-eccentricity orbits, high-mass planets exhibit a broad, nearly flat distribution from circular to highly eccentric orbits.

a , Best-fit beta distributions for transiting warm Jupiters more massive (red) and less massive (blue) than 1.935 M J . The shaded regions represent the 1 σ (darkest), 2 σ and 3 σ (lightest) posteriors on each fit. b , Masses and eccentricities of transiting warm Jupiters. The super-eccentric hot-Jupiter progenitors HD 80606 b and TIC 241249530 b are labelled with upright and inverted stars, respectively. The horizontal dashed line indicates the median mass of the population, which is the threshold used for the fits shown in a . Our results are insensitive to the exact value of the threshold (see Methods ); the median is chosen simply for visualization purposes. Error bars on individual data points indicate the 1 σ uncertainties from the literature.
Although the observed mass–eccentricity correlation may be shaped by several processes, such as collisional eccentricity growth 30 or resonant interactions with the protoplanetary disk 31 , 32 , 33 , the high masses of TIC 241249530 b and HD 80606 b may offer a clue as to the dearth of super-eccentric giant planets. During the high-eccentricity phase of vZLK oscillations, orbital eccentricities can be driven so close to unity that the exoplanet will approach, or even breach, the tidal radius of the host star. Because the tidal radius is inversely proportional to the planet–star mass ratio, lower-mass planets more easily cross this threshold and experience tidal disruption. A relative dearth of low-mass, eccentric progenitors to hot Jupiters is a consistent outcome of simulations of high-eccentricity tidal migration following vZLK oscillations under an equilibrium tide assumption 6 , 7 , 8 . For planets susceptible to chaotic, or diffusive, dynamical tidal evolution, whereby oscillations excited in the planet accelerate orbital decay, this mass dependence is largely erased, as low-mass planets can become decoupled from the perturber before being disrupted 34 . However, chaotic tides facilitate circularization on a much shorter timescale (<100 Myr); these planets will spend very little time with intermediate-period orbits 34 , 35 , 36 . It is possible that only the most massive eccentric giant planets last long enough in this period regime to be represented in the observed sample.
TIC 241249530 b passes through periastron just six hours before each transit, presenting a unique opportunity to observe how an exoplanet atmosphere responds to a rapid, extreme heating event. Temporal variations in exoplanet atmospheres are best explored through studies of planets on eccentric orbits, for which we may see signatures of time-varying irradiation and changing pressure–temperature profiles, such as turbulent surface flows 37 and disequilibrium chemistry 38 , depending on the heat-redistribution timescales. The atmospheres of several eccentric giant planets have been studied 39 , 40 , 41 , but the periastron phase has not been captured in transit for these systems. The orbital geometry of TIC 241249530 b will make such measurements possible for the first time. The planetary atmosphere can also be studied by means of emission measurements during other orbital phases, but the orientation precludes a secondary eclipse at a 6.3 σ confidence.
TESS photometry
TIC 241249530 was observed with TESS 9 from 24 December 2019 to 21 January 2020 (Sector 20) at a 30-min cadence and from 21 December 2022 to 18 January 2023 (Sector 60) at a 2-min cadence. A single transit-like dip (flux depth about 8 parts per thousand) was identified by the TESS Single Transit Planet Candidate Working Group (TSTPC WG) in the Sector 20 Quick Look Pipeline 42 , 43 light curve using a box least-squares search. The TSTPC WG focuses on searching full-frame TESS light curves for isolated transit events and validating and confirming those that are true planets, with the aim of increasing the yield of TESS planets with period >30 days (for example, refs. 44 , 45 , 46 , 47 ). There is no flux centroid motion during the transit event for TIC 241249530 and we identify no other sources brighter than Δ m G = 5 in the target aperture. Although there is flux contamination from two nearby stars with 6 > Δ m G > 5, TIC 241249532 and TIC 241249533, both of which were centred on the same pixel as TIC 241249530 in Sector 20, these are too faint to have been responsible for the observed change in brightness. No notable brightness variations were detected in the Sector 60 light curve. For all subsequent analysis in this work, we rely on the pre-search data conditioned simple aperture photometry 48 , 49 , 50 (PDCSAP) light curve from the Science Processing Operations Center 51 (SPOC) for Sector 60 and the TESS-SPOC 52 light curve for Sector 20 (Extended Data Fig. 1 ).
High-contrast imaging
To verify that the transit signature detected by TESS was indeed associated with TIC 241249530 and not with a nearby star or binary system that was blended in the TESS aperture, we used the NN-EXPLORE Exoplanet Stellar Speckle Imager (NESSI) 10 on the WIYN 3.5-m telescope at Kitt Peak National Observatory to conduct high-spatial-resolution observations of the target on 21 April 2021. A sequence of 1,000 40-ms exposures was taken in the 832-nm and 562-nm narrow-band filters simultaneously with the red and blue NESSI cameras, respectively. These diffraction-limited exposures were used to reconstruct high-contrast images (Extended Data Fig. 2 ) following the steps outlined in ref. 53 . The achieved 5 σ contrast limits are sufficient to rule out the presence of faint stellar companions and background sources with Δmag 562 < 3.3 and Δmag 832 < 3.7 at a separation of 0.2″ and Δmag 562 < 3.9 and Δmag 832 < 4.8 at a separation of 1″.
Ground-based photometric observations
We used the Unistellar Network, a collaboration of citizen scientists using Unistellar telescopes 54 in support of astronomical research, to observe TIC 241249530 from locations in Japan, Europe and the United States in search for transit signatures in March 2023. Observations were taken at various times from 7 to 19 March 2023, when the companion orbital period and transit ephemeris were still highly uncertain. After removing off-target and saturated frames, we calibrated the remaining images, binned them in sets of 15–30 to amplify the signal-to-noise ratio (S/N) and performed differential photometry 55 , 56 . No signatures of statistical significance were found in the Unistellar data and, based on our subsequent orbit fit, we confirm that none of these observations were taken during the transit.
We observed TIC 241249530 again on 30 August 2023 with the Astrophysical Research Consortium Telescope Imaging Camera (ARCTIC) 16 on the ARC 3.5-m telescope at Apache Point Observatory (APO). Observations were conducted using a beam-shaping diffuser, which creates a stable top-hat point spread function of the star to improve photometric precision 15 . We used the Semrock narrow-band filter (838–876 nm) to avoid atmospheric absorption bands 57 . We began observing when the target rose above an air mass of 4 (altitude ≈ 10°) and continued until 12° morning twilight, collecting a continuous 4.3-h baseline of consecutive 30-s exposures. As the star rose above air mass approximately 1.5, about 2.5 h after the start of the observing sequence, a transit-like decrease in brightness was observed.
To reduce the ARCTIC data, a median-combined master bias image was constructed and subtracted from the individual science frames, which were flat-fielded using dome flat exposures taken at the start of the night. We performed differential aperture photometry on the reduced data using AstroImageJ 58 with a 17-pixel (7.7″) aperture and four reference stars that were carefully selected to minimize the scatter of the out-of-transit flux. Flux uncertainties were calculated following the procedures in refs. 15 , 59 , which account for photon noise from the star and background, detector read noise and air-mass-dependent scintillation noise. We removed exposures flagged by AstroImageJ for approaching the detector saturation limit, as well as exposures taken during intermittent cloud cover that introduced further scatter.
The diffused point spread function of TIC 241249530 overlapped with that of TIC 241249532. Before initiating our ARCTIC observing sequence, we collected several individual exposures without the diffuser in the optical path. We used these data to calculate the relative brightness contributions of the two stars. TIC 241249532 contributes just 0.53% of the total flux in the Semrock bandpass.
Spectroscopic observations
We monitored the RV signal of TIC 241249530 with the NEID spectrograph 11 on the WIYN 3.5-m telescope, collecting measurements on 40 separate nights between 2 September 2021 and 1 March 2024. On all but three of these nights, single exposures were taken, with exposure times ranging from 500 to 1,800 s, depending on the observing conditions. On the night of 30 August 2023, four consecutive 20-min exposures were taken simultaneously with the partial transit as observed with ARCTIC, and on the subsequent night, we secured a pair of measurements separated by an hour. We also obtained a sequence of 15 consecutive 20-min exposures on the night of 12 February 2024; this sequence covered a full transit as well as several measurements before ingress and after egress. We discard two spectra that were taken on nights for which the wavelength calibration was identified to be unreliable, leaving us with 56 high-quality measurements with a median S/N per extracted pixel of 25 at 550 nm. The raw echelle spectra were processed with version 1.3 of the NEID Data Reduction Pipeline (DRP; https://neid.ipac.caltech.edu/docs/NEID-DRP/ ), which produces wavelength-calibrated 1D spectra and then calculates RVs using the cross-correlation function (CCF) method 60 . We also independently calculated the RVs from the calibrated 1D spectra using a modified version of the SpEctrum Radial Velocity AnaLyser (SERVAL) template-matching algorithm 61 , 62 that has been optimized for NEID spectra as described by ref. 63 . The SERVAL RVs were calculated using the central 7,000 pixels of 79 orders centred between 4,010 and 8,400 Å (order indices 20 to 100, corresponding to echelle orders 153 to 73). The template-matching results outperform the CCF-based RVs from the NEID DRP, with median single measurement precisions of σ RV,SERVAL = 6.3 m s −1 and σ RV,DRP = 7.9 m s −1 , so we chose to use the SERVAL RVs for the analysis performed in this work.
Further RV measurements were taken with the Habitable-zone Planet Finder (HPF) spectrograph 12 , which is on the Hobby–Eberly Telescope (HET) 64 , 65 at McDonald Observatory, and the HARPS-N spectrograph, mounted on Telescopio Nazionale Galileo (TNG) in La Palma, as TIC 241249530 approached periastron in March 2023. Six HPF observations were made between 6 and 31 March 2023, for which each observation consisted of two consecutive 945-s exposures with a median nightly binned S/N per extracted pixel of 137 at 1,000 nm. These data were processed using the HxRGproc 66 and barycorrpy 67 packages and the RVs were calculated using a version of SERVAL that has been modified for HPF 68 , 69 . We achieve a median RV measurement precision of 15.0 m s −1 . We also observed the target five times with HARPS-N between 7 and 18 March 2023, with an exposure time of 3,300 s and a mean (min, max) S/N of 55 (37, 75). We reduced the data with the offline version of the HARPS-N data-reduction software through the Yabi web interface 70 installed at the Italian Center for Astronomical Archives Data Center. To extract the RVs, we used a G2 mask template and obtained a CCF width of 9.9 km s −1 , with an average precision of 0.1 km s −1 . The median resulting RV measurement precision is 3.4 m s −1 . We show the complete RV time series from NEID, HPF and HARPS-N in Extended Data Fig. 3 .
Stellar characterization of TIC 241249530
To determine the stellar atmospheric parameters of TIC 241249530, we analysed the out-of-transit NEID spectra collected before September 2023 (cumulative S/N ≈ 100 at 550 nm) using the iSpec 71 , 72 Python package to perform synthetic spectral fitting. We used the SPECTRUM radiative transfer code 73 , MARCS atmospheric models 74 , solar abundances from 3D hydrodynamic models 75 and the sixth version of the Gaia ESO survey (GES) atomic line list 76 . The microturbulence velocity was treated as a free parameter to allow for flexibility in accounting for small-scale motions in the stellar atmosphere. Macroturbulence was determined using an empirical relation, making use of established correlations with other stellar properties 77 . To streamline the fitting, we restricted the analysis to specific spectral regions from 480 to 680 nm, encompassing the wing segments of the Hα, Hβ and Mg I triplet lines, which are sensitive to T eff and log g , and the Fe I and Fe II lines, which provide precise constraints on [Fe/H] and v sin i ⋆ . We minimize the difference between the synthetic and input spectra by applying the nonlinear least-squares Levenberg–Marquardt fitting algorithm, using constraints from the aforementioned models and line lists.
The HARPS-N spectra were independently analysed with BACCHUS 78 , using MARCS atmospheric models, the GES atomic line list and the TURBOSPECTRUM radiative transfer code 79 , 80 . For our fit, we constrained T eff by requiring Fe I line abundances to be uncorrelated with their respective excitation potentials in the synthetic spectrum and we constrained log g by requiring ionization balance for the Fe I and Fe II lines. We also required the Fe I line abundances to be uncorrelated with their equivalent widths and the stellar metallicity ([Fe/H]) was calculated as the average of these abundances. The projected rotational velocity was estimated by fitting the broadening of the Fe I lines, accounting for the best-fit microturbulence and assuming the same macroturbulence contribution as in the iSpec analysis. The stellar parameters derived from the NEID and HARPS-N spectra are largely in good agreement (<1 σ ). Discrepancies between the [Fe/H] values and v sin i ⋆ values at the 1.2 σ level probably result from differences between the fitted microturbulence, which is known to exhibit small variations for different fitting methods 72 . We adopt the iSpec T eff , log g , [Fe/H] and v sin i ⋆ for the rest of the analysis in this work.
We performed an analysis of the broadband SED of TIC 241249530 together with the Gaia DR3 parallax following the procedures described in refs. 81 , 82 , 83 . We use JHK S magnitudes from 2MASS 84 , W1–W3 magnitudes from WISE 85 , G BP G RP magnitudes from Gaia 86 , BVgri magnitudes from APASS 87 and the NUV magnitude from GALEX 88 . We also used the Gaia spectrophotometry spanning 0.4–1.0 µm. Altogether, the available photometry spans the full stellar SED over the wavelength range 0.2–10.0 µm. We fit the SED using PHOENIX stellar atmosphere models 89 , with the effective temperature, surface gravity and metallicity set to the spectroscopically determined values. The remaining free parameter is the extinction ( A V ), which we limited to the maximum line-of-sight value of A V = 0.44 mag from galactic dust maps 90 . The resulting fit is shown in Extended Data Fig. 4 . Integrating the unreddened model SED yields the bolometric flux at Earth, F bol = 7.19 ± 0.20 × 10 −10 erg s −1 cm −2 . Taking the F bol and T eff together with the Gaia parallax, we calculate the stellar radius to be R ⋆ = 1.404 ± 0.028 R ⊙ . Also, the stellar mass is inferred using empirical relations 91 , giving M ⋆ = 1.24 ± 0.07 M ⊙ , and we estimate the age to be 3.2 ± 0.5 Gyr by fitting the evolutionary state with the Yonsei–Yale isochrone models 92 . Our reported 0.5-Gyr uncertainty accounts for the uncertainties on each of the inputs to the isochrone fit: effective temperature, surface gravity, metallicity and stellar mass. However, this does not account for systematic uncertainties arising from our choice of stellar models, which can be on the order of 1 Gyr.
The best-fit extinction for our SED model is A V = 0.31 ± 0.02. This large value is supported by a clear detection of interstellar absorption in the Na D doublet and the K I 770 nm lines in the NEID spectra. Both spectroscopic analyses yield T eff values that are substantially hotter than the literature value from Gaia DR3 spectrophotometric analysis 92 , which is consistent with the effect of reddening from dust along the line of sight to the star.
Using the projected rotational velocity and stellar radius, we place an upper limit on the rotation period of \({16.9}_{-2.6}^{+3.8}\,{\rm{days}}\) . We attempt to make a more precise measurement of the rotation period to determine the stellar inclination, but the existing data are insufficient. An analysis of the TESS light curves using the TESS Systematics-Insensitive Periodogram package 93 shows no notable photometric modulation on timescales shorter than the length of each individual sector. We also examine archival photometry of the star from the WASP survey 94 . These data consist of 2,178 measurements on 33 nights, with two isolated epochs in April 2006 and March 2007, and the remaining data covering October 2007 to March 2008. In spite of the substantially longer baseline than TESS, a Lomb–Scargle periodogram analysis of the WASP measurements reveals no notable peaks besides the half-day, one-day and two-day sampling aliases. The lack of photometric modulation is reflected in the spectroscopic data as well; we do not detect periodic variation in the activity-sensitive Ca II H & K, Na I or Hα spectral lines as measured by the NEID DRP. Also, there is no emission in the Ca II H & K line cores in the NEID and HARPS-N spectra, suggesting that the star is chromospherically quiet.
Stellar characterization of TIC 241249532
TIC 241249530 shares a common parallax and proper motion with TIC 241249532 as measured by Gaia, and the two stars are separated on the sky by 4.930 ± 0.104″ (ref. 86 ). The probability of a chance alignment between TIC 241249530 and TIC 241249532 is R = 9.73 × 10 −5 (ref. 95 ), suggesting that the pair is indeed gravitationally bound. Gaia’s photometric measurements of TIC 241249532 place it firmly along the main sequence. We do not perform an independent SED analysis on this star but instead estimate its mass using empirical mass–luminosity relations 96 , 97 . We calculate the mass to be 0.453 ± 0.012 times that of the Sun based on the 2MASS K s -band magnitude and 0.400 ± 0.016 times that of the Sun based on the Gaia G RP -band magnitude. The stellar mass, coordinates and broadband photometry are given in Extended Data Table 1 .
On the basis of the weighted mean of the Gaia parallax measurements for the system, the on-sky separation corresponds to a projected physical separation of 1,664.00 ± 10.85 au . The relative motions of these two stars are not constrained well enough by Gaia to meaningfully estimate an orbital solution. However, as an effort to quantify the dynamical impact of TIC 241249532 in our analysis, we simulated 10 million orbits sampled randomly in phase, uniformly in cos i and thermally ( f ( e ) = 2 e ) in eccentricity. We determine the orbital period of the system to be >10,000 years, with a peak in frequency at 35,000 years. Long-period stellar companions such as this can directly bias RV analyses of exoplanets in the form of a linear RV slope. However, our simulations show that TIC 241249532 probably induces a linear trend in the observed RVs of TIC 241249530 at the level of just 1 cm s −1 year −1 , with 99% of our orbits returning slopes <30 cm s −1 year −1 . The amplitude of this signal is small compared with the km-s −1 -level variations induced by TIC 241249530 b and we therefore do not include it as an extra body in our joint fitting.
Joint transit + RV analysis
We use the exoplanet software package 98 to fit a transit model and a Keplerian orbit with Rossiter–McLaughlin perturbations to the observed photometric and RV signals for TIC 241249530. The exoplanet package relies on starry 99 , 100 and the underlying analytic models from ref. 101 to fit the transits, and the orbital parameter posteriors are sampled using the PyMC3 Hamiltonian Monte Carlo package 102 . The orbit model consists of a full Keplerian with tight Gaussian priors on the orbital period, P , and time of conjunction, T 0 , broad uniform priors on the exoplanet mass, M p , transit impact parameter, b , and transit depth, δ , and Gaussian priors on the stellar mass, M ⋆ , and radius, R ⋆ . We reparameterize the eccentricity, e , and argument of periastron, ω , as \(\sqrt{e}\sin \omega \) and \(\sqrt{e}\cos \omega \) and we sample these on the unit disk. We do not impose an extra eccentricity prior, as the global warm-Jupiter eccentricity distribution is not well constrained 103 . Separate quadratic limb-darkening coefficients, reparameterized as q 1 and q 2 as in ref. 104 , are used for each instrument for which in-transit observations were taken (TESS, ARCTIC, NEID). For the RV data, we fit individual zero-point offset terms ( σ ) and jitter terms ( γ ) for each instrument, splitting the NEID RVs into two separate datasets before and after the instrument restart. Dilution terms are included for both TESS and ARCTIC, as both transit measurements suffered from flux contamination. The TESS data products already account for dilution, but previous works have demonstrated that these results are susceptible to overcorrection 105 , 106 , so we allow the TESS dilution term to float uniformly from 0.1 to 1.2. For ARCTIC, we fix the dilution to be 0.9947 based on the out-of-transit data for which the target was well resolved from its companion. To model the Rossiter–McLaughlin signal, we adopt the formalism of ref. 107 along with their prior distributions for the Gaussian line dispersion parameter, β , the Lorentzian line dispersion parameter, γ , and macroturbulence, ζ . We place a Gaussian prior on the projected stellar rotational velocity, v sin i ⋆ , and a uniform prior on the projected spin–orbit misalignment, λ . The prior distributions and posterior results for all of these fit parameters, as well as for some derived values, are given in Extended Data Table 2 .
Stellar obliquity
The stellar obliquity, ψ , is related to the projected obliquity, λ , by
Here i ⋆ is the inclination of the stellar spin axis and i is the inclination of the exoplanet orbit. We cannot directly calculate ψ because the stellar inclination is not known. Instead, assuming that the stellar inclination is drawn from an isotropic distribution, uniform in cos i ⋆ , we use the above equation to determine the possible values of ψ and their relative probabilities. For our derived posteriors on λ and i , we find that the orbit is indeed retrograde (that is, ψ > 90°) at 99.5% confidence in this scenario, and we calculate the obliquity to be \(\psi ={141}_{-24}^{{+15}^{\circ }}\) . This value is consistent with expectations for vZLK-driven migration; simulations show that the final obliquity can be as large as 180° for systems such as this 7 . This is not definitive proof of the formation history, however, as retrograde orbits such as this can also be produced through planet–planet interactions 108 , 109 . Regardless, we warn that our result is strongly dependent on the naive assumption of an isotropic stellar inclination distribution, which is not always valid 110 , 111 .
Dynamical history—analytic constraints
The high eccentricity and tight orbit of TIC 241249530 b and the presence of the distant stellar binary companion indicate a likely history of high-eccentricity migration driven by vZLK oscillations and tidal dissipation. To determine how this formation channel could have delivered the exoplanet to its current orbit, we first identify a set of initial conditions consistent with the present-day architecture of the system. We work in the context of the secular approximation for the evolution of hierarchical triple configurations 112 .
The planet is at present close enough to the primary star such that short-range forces—general relativity (GR), tides and rotational distortions—have quenched any vZLK oscillations driven by the companion. We calculate the semimajor axis at which this quenching occurs by assuming that GR dominates the short-range forces and examining the ratio between the timescale of GR precession of the inner orbit and the timescale for vZLK oscillations. To leading order (quadrupole limit), this ratio is 113
in which M ⋆ is the mass of the primary star; M p , a and e are the mass, semimajor axis and eccentricity of the planet; and M 2 , a 2 and e 2 are the mass, semimajor axis and eccentricity of the binary companion. For the exoplanet and primary-star parameters, we adopt the median posteriors from our joint fit. For the binary companion, we set M 2 = 0.453 M ⊙ , a 2 = 1,664 au and e 2 = 0.5. If the planet started with a low initial eccentricity of e = 0.1, vZLK oscillations would have started only if the initial semimajor axis of the planet was a i > 4.2 au , for which this value is calculated by setting t GR / t quad = 1.
We can now constrain the initial eccentricity by requiring that the periastron distance of the first vZLK oscillation was sufficiently small to trigger efficient tidal dissipation. In particular, in the quadrupole limit, the quantity \({a}_{{\rm{f}}}\equiv a\left(1-{e}_{\max }^{2}\right)\) is approximately conserved throughout the tidal migration, as the orbital angular momentum is conserved both during episodes of maximum eccentricity, as well as after vZLK oscillations have been quenched. Here e max indicates the maximum eccentricity reached during a vZLK oscillation and a f is equal to the final semimajor axis once the orbit has fully circularized. If a f is taken to be conserved, the maximum eccentricity of the initial vZLK oscillation must have been e i,max > 0.9947.
Exciting an eccentricity this high on the initial vZLK oscillation must have required a substantial initial inclination, I i , between the orbit of the planet and that of the binary companion. We derive a lower bound on I i using the following equation from ref. 112 :
Here \({j}_{{\rm{i}},\min }=\sqrt{1-{e}_{{\rm{i}},\max }^{2}}\) and we have assumed GR perturbations to be dominant over those from tidal and rotational distortion. The dimensionless quantity ε GR measures the ‘strength’ of perturbations from GR relative to those of the binary companion and it is defined as
Extended Data Fig. 5 shows the required initial inclination between the planetary and binary orbital planes and the resulting initial maximum eccentricity with respect to the initial semimajor axis of the planet. Although vZLK oscillations are present when a i > 4.2 au , not all values above this threshold yield defined values for I i because the short-range forces are too strong for the planet to reach the required high initial eccentricity unless the initial semimajor axis exceeds a i > 7.0 au . The maximum eccentricity of the initial vZLK cycle must have been e i,max > 0.9947 to generate the present-day semimajor axis and eccentricity. Attaining a maximum eccentricity this large is only possible with a nearly polar initial inclination between the orbit planes of the planet and the binary companion. We find that the initial inclination I i > 86.8° for a i > 7.0 au . Altogether, these results indicate that it is possible to reach the present-day parameters of the system if the planet started beyond a i > 7.0 au and the binary companion started on an orbit nearly perpendicular to that of the planet.
Dynamical history—simulations
We now use our derived constraints on the initial orbital conditions to explore the planetary orbital evolution through numerical simulations. We conduct integrations of the secular equations of motion for TIC 241429530 through KozaiPy, a publicly available software package that simulates hierarchical three-body systems ( https://github.com/djmunoz/kozaipy ). The equations of motion are provided in ref. 3 . We adopt initial values of a i = 10 au and e i = 0.1, considering the minimum semimajor axis necessary for vZLK oscillations to be present. We consider perturbations to the octupole order and also account for tidal evolution in the constant-time-lag model of equilibrium tides 114 . Tidal parameters are adjusted so that the system reaches its present-day orbital parameters at an age of 3 Gyr, approximately equal to the derived age of the system. Specifically, the Love number of the planet is set to k 2 = 0.25 and its viscous timescale is set to t v = 0.01 days.
The simulation results are presented in Extended Data Fig. 6 . They indicate the presence of vZLK oscillations that trigger periods of very large eccentricities. At the times that the periapse distance is minimized, tidal dissipation is strong and the semimajor axis shrinks. Eventually, the semimajor axis becomes small enough that the vZLK oscillations are suppressed owing to short-range forces and the planet decouples from the binary companion. After the vZLK oscillations are quenched, the mutual inclination is approximately conserved and the eccentricity of the planet slowly damps owing to continued tidal dissipation. We observe that there is an instant in time at which the eccentricity and semimajor axis of the planet are very close to the present-day values. We also note that the value of \(a(1-{e}_{\max }^{2})\) is conserved during episodes of maximum eccentricity of each individual vZLK cycle, ranging within only a few percent of the average value of \(a(1-{e}_{\max }^{2})\) . According to this simulation, continued dissipation will cause the planet to reach a circular orbit in about a billion years. Altogether, this simulation provides a plausible proof of concept of the system’s dynamical history of coupled vZLK oscillations and tidal migration. We suggest future work on the system to explore the role that dynamical tides might have played in its formation.
Modelling the transiting-warm-Jupiter eccentricity distribution
To explore the relation between exoplanet mass and eccentricity for warm Jupiters, or intermediate-period giant planets, we start with the sample of all transiting exoplanets with masses between 0.3 and 15 times that of Jupiter and orbital periods between 10 and 365 days. For each system in this sample, we adopt the most up-to-date mass and eccentricity constraints for which the eccentricity was fit as a free parameter when solving for the orbit. We discard four planets for which all literature solutions assumed a circular orbit with the eccentricity fixed at 0. All of these planets are less than 1.3 Jupiter masses. We also remove two planets in P-type circumbinary orbits, as the dynamical environments of these systems are expected to differ from those of planets orbiting single stars 115 , 116 . Our sample differs from that analysed in ref. 41 , which draws from the RV planet sample and thus uses projected planet mass ( M p sin i ) instead of true mass. By restricting our analysis to transiting exoplanets, we ensure that the measured masses are not degenerate with orbital inclination. This approach also mitigates the susceptibility of our results to detection biases, as the completeness fractions of transit surveys should be largely insensitive to exoplanet mass in the Jupiter-sized-planet regime.
The median mass of our sample is 1.935 Jupiter masses. We divide the sample into two groups of equal size, placing planets more massive than the median into one group and planets less massive than the median into the other group. The population-level eccentricity distribution of each group is then modelled in PyMC 117 using a hierarchical Bayesian framework. For our model, we adopt a beta distribution with two hyperparameters, θ = { µ , κ }, in which µ describes the mean of the distribution and 1/ κ describes its variance. These hyperparameters represent a reparameterization of the standard beta distribution parameters α and β , in which α = µκ and β = (1 − µ ) κ . A beta distribution is chosen for its flexibility in shape and because it is naturally bounded between 0 and 1. We adopt a uniform hyperprior for µ ~ U (0, 1) and a log-normal hyperprior for log κ ~ N (0, 3). These choices reduce the impact of hyperprior choices on the inference results, especially when the sample size is small, as is the case here 118 . The best-fit distributions are shown in Fig. 3 and the resulting hyperparameters are \({\mu }_{{\rm{low}}}={0.18}_{-0.03}^{+0.04}\) , \(\log {\kappa }_{{\rm{low}}}={1.23}_{-0.29}^{+0.27}\) , \({\mu }_{{\rm{high}}}={0.44}_{-0.05}^{+0.05}\) and \(\log {\kappa }_{{\rm{high}}}={0.91}_{-0.24}^{+0.22}\) . The mean values, µ , of the eccentricity distributions of low-mass and high-mass transiting warm Jupiters differ by 4.2 σ . To assess the robustness of this result, we repeated the process for mass cutoffs between 1 Jupiter mass and 2.7 Jupiter masses. These bounds were chosen such that the size ratio of the two groups does not exceed 2:1. At each cutoff, we ran 1,000 trials, drawing the planet masses from asymmetric Gaussian distributions with means and widths determined by their literature values and uncertainties. For all mass-cutoff values over this range, the mean values of the two eccentricity distributions differ by 3–5 σ .
Data availability
The TESS data products referenced and analysed in this work are publicly available through the Mikulski Archive for Space Telescopes (MAST) at https://exo.mast.stsci.edu/ . The raw NEID, HPF and HARPS-N spectra are available on request. Source data are provided with this paper.
Code availability
AstroImageJ is publicly available and can be downloaded at https://www.astro.louisville.edu/software/astroimagej/installation_packages/ . The exoplanet software package is open source and public and can be downloaded at https://github.com/exoplanet-dev/exoplanet . The PyMC software package is open source and public and can be downloaded at https://github.com/pymc-devs/pymc .
Dawson, R. I. & Johnson, J. A. Origins of hot Jupiters. Annu. Rev. Astron. Astrophys. 56 , 175–221 (2018).
Article ADS Google Scholar
Holman, M., Touma, J. & Tremaine, S. Chaotic variations in the eccentricity of the planet orbiting 16 Cygni B. Nature 386 , 254–256 (1997).
Article ADS CAS Google Scholar
Fabrycky, D. & Tremaine, S. Shrinking binary and planetary orbits by Kozai cycles with tidal friction. Astrophys. J. 669 , 1298–1315 (2007).
Naef, D. et al. HD 80606 b, a planet on an extremely elongated orbit. Astron. Astrophys. 375 , L27–L30 (2001).
Wu, Y. & Murray, N. Planet migration and binary companions: the case of HD 80606b. Astrophys. J. 589 , 605–614 (2003).
Petrovich, C. Steady-state planet migration by the Kozai-Lidov mechanism in stellar binaries. Astrophys. J. 799 , 27 (2015).
Anderson, K. R., Storch, N. I. & Lai, D. Formation and stellar spin-orbit misalignment of hot Jupiters from Lidov–Kozai oscillations in stellar binaries. Mon. Not. R. Astron. Soc. 456 , 3671–3701 (2016).
Muñoz, D. J., Lai, D. & Liu, B. The formation efficiency of close-in planets via Lidov–Kozai migration: analytic calculations. Mon. Not. R. Astron. Soc. 460 , 1086–1093 (2016).
Ricker, G. R. et al. Transiting Exoplanet Survey Satellite (TESS). J. Astron. Telesc. Instrum. Syst. 1 , 014003 (2015).
Scott, N. J. et al. The NN-explore Exoplanet Stellar Speckle Imager: instrument description and preliminary results. Publ. Astron. Soc. Pac. 130 , 054502 (2018).
Schwab, C. et al. Design of NEID, an extreme precision Doppler spectrograph for WIYN. Proc. SPIE 9908 , 99087H (2016).
Google Scholar
Mahadevan, S. et al. The Habitable-zone Planet Finder: a stabilized fiber-fed NIR spectrograph for the Hobby-Eberly Telescope. Proc. SPIE 8446 , 84461S (2012).
Article Google Scholar
Cosentino, R. et al. Harps-N: the new planet hunter at TNG. Proc. SPIE 8446 , 84461V (2012).
Peluso, D. O. et al. The Unistellar Exoplanet Campaign: citizen science results and inherent education opportunities. Publ. Astron. Soc. Pac. 135 , 015001 (2023).
Stefánsson, G. et al. Toward space-like photometric precision from the ground with beam-shaping diffusers. Astrophys. J. 848 , 9 (2017).
Huehnerhoff, J. et al. Astrophysical Research Consortium Telescope Imaging Camera (ARCTIC) facility optical imager for the Apache Point Observatory 3.5m telescope. Proc. SPIE 9908 , 99085H (2016).
Albrecht, S. H., Dawson, R. I. & Winn, J. N. Stellar obliquities in exoplanetary systems. Publ. Astron. Soc. Pac. 134 , 082001 (2022).
von Zeipel, H. Sur l’application des séries de M. Lindstedt à l'étude du mouvement des comètes périodiques. Astron. Nachr. 183 , 345 (1910).
Lidov, M. L. The evolution of orbits of artificial satellites of planets under the action of gravitational perturbations of external bodies. Planet. Space Sci. 9 , 719–759 (1962).
Kozai, Y. Secular perturbations of asteroids with high inclination and eccentricity. Astron. J 67 , 591–598 (1962).
Article ADS MathSciNet Google Scholar
Dong, J. et al. TOI-3362b: a proto hot Jupiter undergoing high-eccentricity tidal migration. Astrophys. J. Lett. 920 , L16 (2021).
Barbieri, M. et al. HD 17156b: a transiting planet with a 21.2-day period and an eccentric orbit. Astron. Astrophys. 476 , L13–L16 (2007).
Santerne, A. et al. SOPHIE velocimetry of Kepler transit candidates. XII. KOI-1257 b: a highly eccentric three-month period transiting exoplanet. Astron. Astrophys. 571 , A37 (2014).
Dawson, R. I., Murray-Clay, R. A. & Johnson, J. A. The photoeccentric effect and proto-hot Jupiters. III. A paucity of proto-hot Jupiters on super-eccentric orbits. Astrophys. J. 798 , 66 (2015).
Jackson, J. M. et al. Statistical analysis of the dearth of super-eccentric Jupiters in the Kepler sample. Astron. J 165 , 82 (2023).
Socrates, A. et al. Super-eccentric migrating Jupiters. Astrophys. J. 750 , 106 (2012).
Butler, R. P. et al. Catalog of nearby exoplanets. Astrophys. J. 646 , 505–522 (2006).
Ford, E. B. & Rasio, F. A. Origins of eccentric extrasolar planets: testing the planet-planet scattering model. Astrophys. J. 686 , 621–636 (2008).
Wright, J. T. et al. Ten new and updated multiplanet systems and a survey of exoplanetary systems. Astrophys. J. 693 , 1084–1099 (2009).
Frelikh, R. et al. Signatures of a planet–planet impacts phase in exoplanetary systems hosting giant planets. Astrophys. J. Lett. 884 , L47 (2019).
Papaloizou, J. C. B., Nelson, R. P. & Masset, F. Orbital eccentricity growth through disc-companion tidal interaction. Astron. Astrophys. 366 , 263–275 (2001).
Goldreich, P. & Sari, R. Eccentricity evolution for planets in gaseous disks. Astrophys. J. 585 , 1024–1037 (2003).
Romanova, M. M. et al. Eccentricity growth of massive planets inside cavities of protoplanetary discs. Mon. Not. R. Astron. Soc. 523 , 2832–2849 (2023).
Vick, M., Lai, D. & Anderson, K. R. Chaotic tides in migrating gas giants: forming hot and transient warm Jupiters via Lidov–Kozai migration. Mon. Not. R. Astron. Soc. 484 , 5645–5668 (2019).
ADS CAS Google Scholar
Wu, Y. Diffusive tidal evolution for migrating hot Jupiters. Astron. J 155 , 118 (2018).
Rozner, M. et al. Inflated eccentric migration of evolving gas giants I – accelerated formation and destruction of hot and warm Jupiters. Astrophys. J. 931 , 10 (2022).
Langton, J. & Laughlin, G. Hydrodynamic simulations of unevenly irradiated Jovian planets. Astrophys. J. 674 , 1106–1116 (2008).
Mayorga, L. C. et al. Variable irradiation on 1D cloudless eccentric exoplanet atmospheres. Astrophys. J. 915 , 41 (2021).
Laughlin, G. et al. Rapid heating of the atmosphere of an extrasolar planet. Nature 457 , 562–564 (2009).
Article ADS CAS PubMed Google Scholar
Lewis, N. K. et al. Orbital phase variations of the eccentric giant planet HAT-P-2b. Astrophys. J. 766 , 95 (2013).
de Wit, J. et al. Direct measure of radiative and dynamical properties of an exoplanet atmosphere. Astrophys. J. Lett. 820 , L33 (2016).
Huang, C. X. et al. Photometry of 10 million stars from the first two years of TESS full frame images: part I. Res. Notes Am. Astron. Soc. 4 , 204 (2020).
ADS Google Scholar
Huang, C. X. et al. Photometry of 10 million stars from the first two years of TESS full frame images: part II. Res. Notes Am. Astron. Soc. 4 , 206 (2020).
Gupta, A. F. et al. A high-eccentricity warm Jupiter orbiting TOI-4127. Astron. J 165 , 234 (2023).
Harris, M. et al. Separated twins or just siblings? A multiplanet system around an M dwarf including a cool sub-Neptune. Astrophys. J. Lett. 959 , L1 (2023).
Mann, C. R. et al. Giant Outer Transiting Exoplanet Mass (GOT ‘EM) Survey. III. Recovery and confirmation of a temperate, mildly eccentric, single-transit Jupiter orbiting TOI-2010. Astron. J 166 , 239 (2023).
Mireles, I. et al. TOI-4600 b and c: two long-period giant planets orbiting an early K dwarf. Astrophys. J. Lett. 954 , L15 (2023).
Smith, J. C. et al. Kepler Presearch Data Conditioning II - a Bayesian approach to systematic error correction. Publ. Astron. Soc. Pac. 124 , 1000 (2012).
Stumpe, M. C. et al. Kepler Presearch Data Conditioning I—architecture and algorithms for error correction in Kepler light curves. Publ. Astron. Soc. Pac. 124 , 985 (2012).
Stumpe, M. C. et al. Multiscale systematic error correction via wavelet-based bandsplitting in Kepler data. Publ. Astron. Soc. Pac. 126 , 100 (2014).
Jenkins, J. M. et al. The TESS science processing operations center. Proc. SPIE 9913 , 99133E (2016).
Caldwell, D. A. et al. TESS science processing operations center FFI target list products. Res. Notes Am. Astron. Soc. 4 , 201 (2020).
Howell, S. B. et al. Speckle camera observations for the NASA Kepler Mission Follow-up Program. Astron. J 142 , 19 (2011).
Marchis, F. et al. Unistellar eVscopes: smart, portable, and easy-to-use telescopes for exploration, interactive learning, and citizen astronomy. Acta Astronaut. 166 , 23–28 (2020).
Dalba, P. A. & Muirhead, P. S. No timing variations observed in third transit of snow-line exoplanet Kepler-421b. Astrophys. J. Lett. 826 , L7 (2016).
Dalba, P. A. et al. Kepler transit depths contaminated by a phantom star. Astron. J 153 , 59 (2017).
Stefánsson, G. et al. Extreme precision photometry from the ground with beam-shaping diffusers for K2, TESS, and beyond. Proc. SPIE 10702 , 1070250 (2018).
Collins, K. A. et al. AstroImageJ: image processing and photometric extraction for ultra-precise astronomical light curves. Astron. J 153 , 77 (2017).
Stefánsson, G. et al. Diffuser-assisted photometric follow-up observations of the Neptune-sized planets K2-28b and K2-100b. Astron. J 156 , 266 (2018).
Baranne, A. et al. ELODIE: a spectrograph for accurate radial velocity measurements. Astron. Astrophys. Suppl. Ser. 119 , 373–390 (1996).
Zechmeister, M. et al. Spectrum radial velocity analyser (SERVAL). High-precision radial velocities and two alternative spectral indicators. Astron. Astrophys. 609 , A12 (2018).
Anglada-Escudé, G. & Butler, R. P. The HARPS-TERRA project. I. Description of the algorithms, performance, and new measurements on a few remarkable stars observed by HARPS. Astrophys. J. Suppl. Ser. 200 , 15 (2012).
Stefánsson, G. et al. The warm Neptune GJ 3470b has a polar orbit. Astrophys. J. Lett. 931 , L15 (2022).
Ramsey, L. W. et al. Early performance and present status of the Hobby-Eberly Telescope. Proc. SPIE 3352 , 34–42 (1998).
Hill, G. J. et al. The HETDEX instrumentation: Hobby–Eberly telescope wide-field upgrade and VIRUS. Astron. J 162 , 298 (2021).
Ninan, J. P. et al. The Habitable-Zone Planet Finder: improved flux image generation algorithms for H2RG up-the-ramp data. Proc. SPIE 10709 , 107092U (2018).
Kanodia, S. & Wright, J. Python leap second management and implementation of precise barycentric correction (barycorrpy). Res. Notes Am. Astron. Soc. 2 , 4 (2018).
Metcalf, A. J. et al. Stellar spectroscopy in the near-infrared with a laser frequency comb. Optica 6 , 233–239 (2019).
Stefánsson, G. et al. A Neptune-mass exoplanet in close orbit around a very low-mass star challenges formation models. Science 382 , 1031–1035 (2023).
Article ADS PubMed Google Scholar
Hunter, A. A. et al. Yabi: an online research environment for grid, high performance and cloud computing. Source Code Biol. Med. 7 , 1 (2012).
Article PubMed PubMed Central Google Scholar
Blanco-Cuaresma, S. et al. Determining stellar atmospheric parameters and chemical abundances of FGK stars with iSpec. Astron. Astrophys. 569 , A111 (2014).
Blanco-Cuaresma, S. Modern stellar spectroscopy caveats. Mon. Not. R. Astron. Soc. 486 , 2075–2101 (2019).
Gray, R. O. & Corbally, C. J. The calibration of MK spectral classes using spectral synthesis. 1: the effective temperature calibration of dwarf stars. Astron. J 107 , 742–746 (1994).
Gustafsson, B. et al. A grid of MARCS model atmospheres for late-type stars. I. Methods and general properties. Astron. Astrophys. 486 , 951–970 (2008).
Grevesse, N., Asplund, M. & Sauval, A. J. The solar chemical composition. Space Sci. Rev. 130 , 105–114 (2007).
Heiter, U. et al. Atomic data for the Gaia-ESO Survey. Astron. Astrophys. 645 , A106 (2021).
Article CAS Google Scholar
Blanco-Cuaresma, S. et al. The Gaia FGK benchmark stars. High resolution spectral library. Astron. Astrophys. 566 , A98 (2014).
Masseron, T., Merle, T., & Hawkins, K., BACCHUS: Brussels Automatic Code for Characterizing High accUracy Spectra. Astrophysics Source Code Library, record ascl:1605.004 (2016).
Alvarez, R. & Plez, B. Near-infrared narrow-band photometry of M-giant and Mira stars: models meet observations. Astron. Astrophys. 330 , 1109–1119 (1998).
Plez, B., Turbospectrum: code for spectral synthesis. Astrophysics Source Code Library, record ascl:1205.004 (2012).
Stassun, K. G. & Torres, G. Eclipsing binary stars as benchmarks for trigonometric parallaxes in the Gaia era. Astron. J 152 , 180 (2016).
Stassun, K. G., Collins, K. A. & Gaudi, B. S. Accurate empirical radii and masses of planets and their host stars with Gaia parallaxes. Astron. J 153 , 136 (2017).
Stassun, K. G. et al. Empirical accurate masses and radii of single stars with TESS and Gaia. Astron. J 155 , 22 (2018).
Cutri, R. M. et al. VizieR Online Data Catalog: 2MASS All-Sky Catalog of Point Sources (Cutri+ 2003). VizieR Online Data Catalog, 2246, II/246 (2003).
Wright, E. L. et al. The Wide-field Infrared Survey Explorer (WISE): mission description and initial on-orbit performance. Astron. J 140 , 1868–1881 (2010).
Gaia Collaboration, et al. Gaia Data Release 3. A golden sample of astrophysical parameters. Astron. Astrophys. 674 , A39 (2023).
Henden, A. A. et al. APASS Data Release 10. American Astronomical Society Meeting Abstracts #232, 223.06 (2018).
Martin, D. C. et al. The Galaxy Evolution Explorer: a space ultraviolet survey mission. Astrophys. J. 619 , L1–L6 (2005).
Husser, T.-O. et al. A new extensive library of PHOENIX stellar atmospheres and synthetic spectra. Astron. Astrophys. 553 , A6 (2013).
Schlegel, D. J., Finkbeiner, D. P. & Davis, M. Maps of dust infrared emission for use in estimation of reddening and cosmic microwave background radiation foregrounds. Astrophys. J. 500 , 525–553 (1998).
Torres, G., Andersen, J. & Giménez, A. Accurate masses and radii of normal stars: modern results and applications. Astron. Astrophys. Rev. 18 , 67–126 (2010).
Demarque, P. et al. Y 2 isochrones with an improved core overshoot treatment. Astrophys. J. Suppl. Ser. 155 , 667–674 (2004).
Hedges, C. et al. Systematics-insensitive periodogram for finding periods in TESS observations of long-period rotators. Res. Notes Am. Astron. Soc. 4 , 220 (2020).
Pollacco, D. L. et al. The WASP project and the SuperWASP cameras. Publ. Astron. Soc. Pac. 118 , 1407–1418 (2006).
El-Badry, K., Rix, H.-W. & Heintz, T. M. A million binaries from Gaia eDR3: sample selection and validation of Gaia parallax uncertainties. Mon. Not. R. Astron. Soc. 506 , 2269–2295 (2021).
Mann, A. W. et al. How to constrain your M dwarf. II. The mass–luminosity–metallicity relation from 0.075 to 0.70 solar masses. Astrophys. J. 871 , 63 (2019).
Giovinazzi, M. R. & Blake, C. H. A mass–magnitude relation for low-mass stars based on dynamical measurements of thousands of binary star systems. Astron. J 164 , 164 (2022).
Foreman-Mackey, D. et al. exoplanet: gradient-based probabilistic inference for exoplanet data & other astronomical time series. J. Open Source Softw. 6 , 3285 (2021).
Luger, R. et al. starry: analytic occultation light curves. Astron. J 157 , 64 (2019).
Agol, E., Luger, R. & Foreman-Mackey, D. Analytic planetary transit light curves and derivatives for stars with polynomial limb darkening. Astron. J 159 , 123 (2020).
Mandel, K. & Agol, E. Analytic light curves for planetary transit searches. Astrophys. J. 580 , L171–L175 (2002).
Salvatier, J., Wiecki, T. V., & Fonnesbeck, C. PyMC3: Python probabilistic programming framework. Astrophysics Source Code Library, record ascl:1610.016 (2016).
Dong, J. et al. Warm Jupiters in TESS full-frame images: a catalog and observed eccentricity distribution for year 1. Astrophys. J. Suppl. Ser. 255 , 6 (2021).
Kipping, D. M. Efficient, uninformative sampling of limb darkening coefficients for two-parameter laws. Mon. Not. R. Astron. Soc. 435 , 2152–2160 (2013).
Burt, J. A. et al. TOI-824 b: a new planet on the lower edge of the hot Neptune desert. Astron. J 160 , 153 (2020).
Lin, A. S. J. et al. The unusual M-dwarf Warm Jupiter TOI-1899 b: refinement of orbital and planetary parameters. Astron. J 166 , 90 (2023).
Hirano, T. et al. Improved Modeling of the Rossiter-McLaughlin Effect for Transiting Exoplanets. Astrophys. J. 742 , 69 (2011).
Naoz, S. et al. Hot Jupiters from secular planet–planet interactions. Nature 473 , 187–189 (2011).
Li, G. et al. Eccentricity growth and orbit flip in near-coplanar hierarchical three-body systems. Astrophys. J. 785 , 116 (2014).
Ho, S. & Turner, E. L. The posterior distribution of sin( i ) values for exoplanets with M T sin( i ) determined from radial velocity data. Astrophys. J. 739 , 26 (2011).
Morton, T. D. & Johnson, J. A. Discerning exoplanet migration models using spin–orbit measurements. Astrophys. J. 729 , 138 (2011).
Naoz, S. The eccentric Kozai-Lidov effect and its applications. Annu. Rev. Astron. Astrophys. 54 , 441–489 (2016).
Liu, B., Muñoz, D. J. & Lai, D. Suppression of extreme orbital evolution in triple systems with short-range forces. Mon. Not. R. Astron. Soc. 447 , 747–764 (2015).
Leconte, J. et al. Is tidal heating sufficient to explain bloated exoplanets? Consistent calculations accounting for finite initial eccentricity. Astron. Astrophys. 516 , A64 (2010).
Holman, M. J. & Wiegert, P. A. Long-term stability of planets in binary systems. Astron. J 117 , 621–628 (1999).
Pierens, A. & Nelson, R. P. On the formation and migration of giant planets in circumbinary discs. Astron. Astrophys. 483 , 633–642 (2008).
Wiecki, T. et al. pymc-devs/pymc: v5.0.1. Zenodo https://doi.org/10.5281/zenodo.4603970 (2022).
Dong, J. & Foreman-Mackey, D. A hierarchical Bayesian framework for inferring the stellar obliquity distribution. Astron. J 166 , 112 (2023).
Stassun, K. G. et al. The TESS input catalog and candidate target list. Astron. J 156 , 102 (2018).
Download references
Acknowledgements
A.F.G. thanks B. Dawson and J. Najita for guidance. NEID is financed by the National Aeronautics and Space Administration (NASA) through the Jet Propulsion Laboratory (JPL) by contract 1547612 and the NEID Data Reduction Pipeline is financed through JPL contract 1644767. Funding for this work was partially provided by Research Support Agreements 1646897 and 1679618 administered by JPL. The Center for Exoplanets and Habitable Worlds and the Penn State Extraterrestrial Intelligence Center are supported by the Pennsylvania State University and the Eberly College of Science. This research has made use of the SIMBAD database, operated at Strasbourg astronomical Data Center (CDS), Strasbourg, France, and NASA’s Astrophysics Data System Bibliographic Services. This research was carried out, in part, at JPL, California Institute of Technology, under a contract with NASA (80NM0018D0004). Computations for this research were performed on the Pennsylvania State Universityʼs Institute for Computational and Data Sciences Advanced Cyberinfrastructure (ICDS-ACI). This content is solely the responsibility of the authors and does not necessarily represent the views of the Institute for Computational and Data Sciences. This paper contains data taken with the NEID instrument, which was financed by the NASA-NSF Exoplanet Observational Research (NN-EXPLORE) partnership and built by Pennsylvania State University. NEID is installed on the WIYN telescope, which is operated by the National Science Foundation (NSF)’s National Optical-Infrared Astronomy Research Laboratory, and the NEID archive is operated by the NASA Exoplanet Science Institute at the California Institute of Technology. Some of the observations in this paper made use of the NN-EXPLORE Exoplanet Stellar Speckle Imager (NESSI). NESSI was financed by the NASA Exoplanet Exploration Program and the NASA Ames Research Center. NESSI was built at the Ames Research Center by S. B. Howell, N. Scott, E. P. Horch and E. Quigley. NN-EXPLORE is managed by JPL, California Institute of Technology under contract with NASA. This work includes data collected by the TESS mission, which are publicly available from the Mikulski Archive for Space Telescopes (MAST). Funding for the TESS mission is provided by the NASA Science Mission Directorate. We acknowledge the use of public TESS data from pipelines at the TESS Science Office and at the TESS Science Processing Operations Center. Resources supporting this work were provided by the NASA High-End Computing (HEC) Program through the NASA Advanced Supercomputing (NAS) Division at Ames Research Center for the production of the SPOC data products. This research has made use of the Exoplanet Follow-up Observing Program website, which is operated by the California Institute of Technology, under contract with NASA under the Exoplanet Exploration Program. Some of the data presented in this paper were obtained from MAST. Support for MAST for non-HST data is provided by the NASA Office of Space Science through grant NNX09AF08G and by other grants and contracts. This work has made use of data from the European Space Agency (ESA) mission Gaia, processed by the Gaia Data Processing and Analysis Consortium (DPAC). Funding for the DPAC has been provided by national institutions, in particular the institutions participating in the Gaia Multilateral Agreement. This research has made use of the NASA Exoplanet Archive, which is operated by the California Institute of Technology, under contract with NASA under the Exoplanet Exploration Program. This research was made possible through the use of the AAVSO Photometric All-Sky Survey (APASS), financed by the Robert Martin Ayers Sciences Fund and NSF AST-1412587. We thank N. Leroux for assistance with the Unistellar observations. C.I.C. acknowledges support by NASA Headquarters through an appointment to the NASA Postdoctoral Program at Goddard Space Flight Center, administered by ORAU through a contract with NASA. W.D.C. acknowledges financial support from the NSF through grant AST-2108801. E.P. and I.C. acknowledge financial support from the Agencia Estatal de Investigación of the Ministerio de Ciencia e Innovación MCIN/AEI/10.13039/501100011033 and the ERDF ‘A way of making Europe’ through project PID2021-125627OB-C32 and from the Centre of Excellence ‘Severo Ochoa’ award to the Instituto de Astrofisica de Canarias. C.X.H. thanks the support of the ARC DECRA project DE200101840. G.N. acknowledges research funding from the Ministry of Education and Science programme the ‘Excellence Initiative – Research University’ conducted at the Centre of Excellence in Astrophysics and Astrochemistry of the Nicolaus Copernicus University in Toruń, Poland. T.M. acknowledges financial support from the Spanish Ministry of Science and Innovation (MICINN) through the Spanish State Research Agency, under the Severo Ochoa Program 2020–2023 (CEX2019-000920-S). D.D. acknowledges support from the NASA Exoplanet Research Program grant 18-2XRP18_2-0136 and from the TESS Guest Investigator Program grant 80NSSC23K0769. G. Stefánsson acknowledges support provided by NASA through the NASA Hubble Fellowship grant HST-HF2-51519.001-A awarded by the Space Telescope Science Institute, which is operated by the Association of Universities for Research in Astronomy, Inc., for NASA, under contract NAS5-26555. Based in part on observations at Kitt Peak National Observatory, NSF’s NOIRLab (proposal IDs 2021A-0388, 2021B-0442, 2022A-627532, 2022B-176691, 2023A-383728, 2023A-722344, 2023B-607177 and 2024A-843425; PI: A. Gupta), managed by the Association of Universities for Research in Astronomy (AURA) under a cooperative agreement with the NSF. We thank the WIYN Observing Associates for their support of our NEID observations. We are honoured to be permitted to conduct astronomical research on Iolkam Du’ag (Kitt Peak), a mountain with particular importance to the Tohono O’odham. We also thank Z. Arnold, J. Davis, M. Edwards, J. Ehret, T. Juan, B. Pisarek, A. Rowe, F. Wortman, the Eastern Area Incident Management Team and all of the firefighters and air support crew who fought the recent Contreras Fire and saved Kitt Peak National Observatory. These results are based on observations obtained with the Habitable-zone Planet Finder Spectrograph on the Hobby–Eberly Telescope (HET). The HPF team acknowledges support from NSF grants AST-1006676, AST-1126413, AST-1310885, AST-1517592, AST-1310875, ATI 2009889, ATI-2009982 and AST-2108512 as well as the NASA Astrobiology Institute (NNA09DA76A) in the pursuit of precision radial velocities in the near-infrared. The HPF team also acknowledges support from the Heising-Simons Foundation through grant 2017-0494. The HET is a joint project of the University of Texas at Austin, the Pennsylvania State University, Ludwig-Maximilians-Universitaet Muenchen and Georg-August Universität Göttingen. The HET is named in honour of its principal benefactors, W. P. Hobby and R. E. Eberly. The Texas Advanced Computing Center (TACC) at the University of Texas at Austin provided high-performance computing, visualization and storage resources that have contributed to the results reported in this paper. C.I.C. and S.V. are NASA Postdoctoral Fellows. B.G., R.K., L.L., M.L., M.P., M.S., G. Simard and S.W. are Unistellar Citizen Scientists. G. Stefánsson is a NASA Sagan Fellow.
Author information
Unaffiliated: Bruno Guillet, Rachel Knight, Liouba Leroux, Margaret Loose, Michael Primm, Masao Shimizu, Georges Simard, Stefan Will
Authors and Affiliations
U.S. National Science Foundation National Optical-Infrared Astronomy Research Laboratory (NSF NOIRLab), Tucson, AZ, USA
Arvind F. Gupta, Mark E. Everett, Pipa Fernandez, Eli Golub, Jesus Higuera, Jessica Klusmeyer, Sarah E. Logsdon, Yatrik Patel, Jayadev Rajagopal & Heidi Schweiker
Department of Astronomy and Astrophysics, The Pennsylvania State University, University Park, PA, USA
Arvind F. Gupta, Jessica Libby-Roberts, Megan Delamer, Andrea S. J. Lin, Suvrath Mahadevan, Jason Wright & Donald P. Schneider
Center for Exoplanets and Habitable Worlds, The Pennsylvania State University, University Park, PA, USA
Department of Physics, Massachusetts Institute of Technology, Cambridge, MA, USA
Sarah C. Millholland & Haedam Im
Kavli Institute for Astrophysics and Space Research, Massachusetts Institute of Technology, Cambridge, MA, USA
Center for Computational Astrophysics, Flatiron Institute, New York, NY, USA
Jiayin Dong
Van Vleck Observatory, Astronomy Department, Wesleyan University, Middletown, CT, USA
Jonathan M. Jackson
Instituto de Astrofísica de Canarias (IAC), La Laguna, Tenerife, Spain
Ilaria Carleo, Thomas Masseron, Grzegorz Nowak & Enric Palle
Departamento de Astrofísica, Universidad de La Laguna (ULL), La Laguna, Tenerife, Spain
Department of Physics and Astronomy, University of Pennsylvania, Philadelphia, PA, USA
Mark R. Giovinazzi & Cullen H. Blake
Earth and Planets Laboratory, Carnegie Institution for Science, Washington DC, USA
Shubham Kanodia
Department of Astronomy, Indiana University Bloomington, Bloomington, IN, USA
Xian-Yu Wang
Department of Physics and Astronomy, Vanderbilt University, Nashville, TN, USA
Keivan Stassun
Department of Physics and Astronomy, University of New Mexico, Albuquerque, NM, USA
Diana Dragomir
Penn State Extraterrestrial Intelligence Center, The Pennsylvania State University, University Park, PA, USA
Jason Wright
School of Mathematical and Physical Sciences, Macquarie University, North Ryde, New South Wales, Australia
Jaime A. Alvarado-Montes & Christian Schwab
The Macquarie University Astrophysics and Space Technologies Research Centre, Macquarie University, North Ryde, New South Wales, Australia
Department of Astronomy and Steward Observatory, University of Arizona, Tucson, AZ, USA
Chad Bender & Andrew Monson
Carl Sagan Center, SETI Institute, Mountain View, CA, USA
Douglas Caldwell & Lauren Sgro
NASA Goddard Space Flight Center, Greenbelt, MD, USA
Caleb I. Cañas, Michael W. McElwain & Steven Villanueva
Center for Planetary Systems Habitability, The University of Texas at Austin, Austin, TX, USA
William D. Cochran
McDonald Observatory, The University of Texas at Austin, Austin, TX, USA
Department of Astronomy and Astrophysics, University of California, Santa Cruz, Santa Cruz, CA, USA
Jet Propulsion Laboratory, California Institute of Technology, Pasadena, CA, USA
Samuel Halverson
Physics Department, Hobart and William Smith Colleges, Geneva, NY, USA
Leslie Hebb
Department of Astronomy, Cornell University, Ithaca, NY, USA
Centre for Astrophysics, University of Southern Queensland, Toowoomba, Queensland, Australia
Chelsea X. Huang
Department of Astronomy and Astrophysics, Tata Institute of Fundamental Research, Mumbai, India
Joe P. Ninan
Institute of Astronomy, Faculty of Physics, Astronomy and Informatics, Nicolaus Copernicus University, Toruń, Poland
Grzegorz Nowak
Department of Physics, Lehigh University, Bethlehem, PA, USA
Joshua Pepper
Department of Physics & Astronomy, University of California, Irvine, Irvine, CA, USA
Paul Robertson
Schmidt Sciences, New York, NY, USA
Department of Astrophysical Sciences, Princeton University, Princeton, NJ, USA
Guðmundur Stefánsson
Anton Pannekoek Institute for Astronomy, University of Amsterdam, Amsterdam, The Netherlands
Department of Physics & Astronomy, University of Minnesota Duluth, Duluth, MN, USA
Daniel J. Stevens
NASA Headquarters, Washington DC, USA
John Wisniewski
Department of Physics, Engineering & Astronomy, Stephen F. Austin State University, Nacogdoches, TX, USA
Carl Ziegler
You can also search for this author in PubMed Google Scholar
Contributions
A.F.G. selected TIC 241249530 for ground-based observations, designed and led the NEID, NESSI and HPF observing programmes, performed the transit and radial velocity analysis, coordinated the analysis of the transiting-warm-Jupiter mass–eccentricity distribution and wrote most of the manuscript. S.C.M. and H.I. performed the analysis of the dynamical history and trajectory of TIC 241249530 b and contributed the associated text and figures. J.D. wrote the code to analyse the eccentricity distributions and contributed to the theoretical interpretation of the results. J.M.J. contributed to the theoretical interpretation of the eccentricity distribution results. I.C. helped coordinate the HARPS-N observations. J.L.-R., M.D. and S.M. coordinated and conducted the ARCTIC observations and data processing. M.R.G. contributed to the characterization of TIC 241249532. A.S.J.L. performed the SERVAL analysis of the NEID spectra and S.K. performed the SERVAL analysis of the HPF spectra. X-Y.W. performed the iSpec analysis of the NEID spectra. D.D. coordinated the efforts of the TESS Single Transit Planet Candidate Working Group. K.S. performed the SED analysis. D.J.S. ran an independent analysis of the stellar SED to validate the results. T.M. performed the BACCHUS analysis of the HARPS-N spectra. S.M. and J. Wright contributed to the interpretation of the significance of the TIC 241249530 system in the context of the exoplanet population and to the coordination of follow-up observations. M.E.E. conducted the NESSI observations, generated the reconstructed speckle images and calculated the contrast limits. S.E.L., H.S., E.G., J.H., J.K., P.F. and Y.P. scheduled and executed the NEID observations. J.A.A.-M., C.B., C.H.B., C.I.C., S.H., S.K., A.S.J.L., S.M., M.W.M., A.M., J.P.N., J.R., P.R., A.R., C.S., G. Stefánsson and J. Wright contributed to the design, development and commissioning of the NEID spectrograph and data-reduction software. C.B., W.D.C., S.K., J.P.N., P.R., A.R. and G. Stefánsson contributed to the design, development and commissioning of the HPF spectrograph and data-reduction software. D.P.S. assisted with the coordination of the HPF observations. G.N. and E.P. coordinated and conducted the HARPS-N observations. L.H. contributed to the development and installation of the diffuser used to obtain the ARCTIC observations. L.H. and J. Wisniewski helped coordinate and execute the ARCTIC observations. S.V. developed the pipeline with which the TESS transit signal for TIC 241249530 b was first detected and P.D., J.P. and C.Z. vetted the alerted signals in TESS Sector 20 and helped validate the transit of TIC 241249530 b. C.X.H. developed the Quick Look Pipeline and D.C. developed the TESS-SPOC pipeline. B.G., R.K., L.L., M.L., M.P., M.S. and G. Simard conducted the Unistellar observations and L.S. analysed the Unistellar data.
Corresponding author
Correspondence to Arvind F. Gupta .
Ethics declarations
Competing interests.
The authors declare no competing interests.
Peer review
Peer review information.
Nature thanks Daniel Fabrycky and Artie Hatzes for their contribution to the peer review of this work. Peer reviewer reports are available.
Additional information
Publisher’s note Springer Nature remains neutral with regard to jurisdictional claims in published maps and institutional affiliations.
Extended data figures and tables
Extended data fig. 1 tess light curves for tic 241249530..
a , Sector 20 TESS-SPOC data (30-min cadence). The vertical dashed line marks the best-fit transit midpoint. b , Sector 60 SPOC data (2-min cadence). Error bars on individual points represent 1 σ measurement uncertainties.
Extended Data Fig. 2 Reconstructed NESSI speckle images and 5 σ contrast curves for TIC 241249530.
Observations were taken simultaneously at 562 nm with the blue camera (upper-left inset image) and at 832 nm with the red camera (upper-right inset image). The contrast curves indicate the limiting magnitude difference at which bound or background sources could be detected for separations between 0.2″ and 1.2″.
Extended Data Fig. 3 RV time series for TIC 241249530.
a , RV measurements from NEID (blue), HPF (black) and HARPS-N (gold) and best-fit orbit model (black curve). b , Residuals to the RV orbit fit. Vertical red lines in both panels mark the predicted transit times. The grey-shaded region bounds the 3 σ confidence intervals for the fit. The horizontal axis is in units of days relative to Barycentric Julian Date (BJD) 2457000. Error bars on individual points represent 1 σ measurement uncertainties.
Extended Data Fig. 4 SED for TIC 241249530.
Red symbols represent the observed photometric measurements, for which the vertical error bars represent the 1 σ measurement uncertainties and the horizontal bars represent the effective width of the passband. Blue symbols are the model fluxes from the best-fit PHOENIX atmosphere model (black). The Gaia spectrophotometry is represented as a grey swathe (see also inset plot).
Extended Data Fig. 5 Constraints on the parameters of the first vZLK oscillation for TIC 241249530 b.
a , Constraints on initial inclination. b , Constrains on initial maximum eccentricity. The red region (0 au < a i < 4.2 au ) indicates the absence of vZLK oscillations. The orange region (4.2 au < a i < 7 au ) indicates the presence of vZLK oscillations but with insufficient e i,max to reach the present-day orbit owing to strong short-range forces (primarily GR, with further contributions from tides and rotational distortions). The blue region ( a i > 7 au ) indicates the presence of vZLK oscillations that can drive the planet to reach present-day parameters.
Extended Data Fig. 6 Simulated evolution of the orbit of TIC 241249530 b resulting from high-eccentricity migration driven by vZLK oscillations.
a , Evolution of the eccentricity of the planetary orbit over time. b , Evolution of the mutual inclination between the planet and binary orbits over time. c , Evolution of the semimajor axis, a , and periastron separation, a (1 − e ), of the planetary orbit over time. The vertical red lines at 3.2 Gyr mark the age at which the orbit reaches the present-day conditions ( e = 0.94, a = 0.64 au ). We adopt a i = 10 au , e i = 0.1 for the initial orbital parameters for illustrative purposes.
Supplementary information
Peer review file, source data, source data fig. 1, source data fig. 2, source data fig. 3, rights and permissions.
Open Access This article is licensed under a Creative Commons Attribution 4.0 International License, which permits use, sharing, adaptation, distribution and reproduction in any medium or format, as long as you give appropriate credit to the original author(s) and the source, provide a link to the Creative Commons licence, and indicate if changes were made. The images or other third party material in this article are included in the article’s Creative Commons licence, unless indicated otherwise in a credit line to the material. If material is not included in the article’s Creative Commons licence and your intended use is not permitted by statutory regulation or exceeds the permitted use, you will need to obtain permission directly from the copyright holder. To view a copy of this licence, visit http://creativecommons.org/licenses/by/4.0/ .
Reprints and permissions
About this article
Cite this article.
Gupta, A.F., Millholland, S.C., Im, H. et al. A hot-Jupiter progenitor on a super-eccentric retrograde orbit. Nature (2024). https://doi.org/10.1038/s41586-024-07688-3
Download citation
Received : 19 January 2024
Accepted : 07 June 2024
Published : 17 July 2024
DOI : https://doi.org/10.1038/s41586-024-07688-3
Share this article
Anyone you share the following link with will be able to read this content:
Sorry, a shareable link is not currently available for this article.
Provided by the Springer Nature SharedIt content-sharing initiative
By submitting a comment you agree to abide by our Terms and Community Guidelines . If you find something abusive or that does not comply with our terms or guidelines please flag it as inappropriate.
Quick links
- Explore articles by subject
- Guide to authors
- Editorial policies
Sign up for the Nature Briefing newsletter — what matters in science, free to your inbox daily.

- Harvard Business School →
- Faculty & Research →
- Working Paper
- HBS Working Paper Series
AI Companions Reduce Loneliness
- Format: Print
- | Language: English
- | Pages: 62
About The Author
Julian De Freitas
More from the authors.
- July 1, 2024
- Harvard Business Review (website)
Research: Speed Matters When Companies Respond to Social Issues
- July 16, 2024
- Proceedings of the National Academy of Sciences
Do ‘Black Individuals’ Really Display No Linguistic Markers of Depression?
- Journal of the Association for Consumer Research
How Artificial Intelligence Constrains Human Experience
- Research: Speed Matters When Companies Respond to Social Issues By: Alison Wood Brooks, Jimin Nam, Maya Balakrishnan and Julian De Freitas
- Do ‘Black Individuals’ Really Display No Linguistic Markers of Depression? By: Julian De Freitas
- How Artificial Intelligence Constrains Human Experience By: A. Valenzuela, S. Puntoni, D. Hoffman, N. Castelo, J. De Freitas, B. Dietvorst, C. Hildebrand, Y.E. Huh, R. Meyer, M. Sweeney, S. Talaifar, G. Tomaino and K. Wertenbroch

COMMENTS
The initial measurement of Jupiter's gravity will inform interior models with implications for the extent, existence, and mass of Jupiter's core. The magnitude of the observed magnetic field observed was 7.766 G, almost twice as strong as expected. More results from Juno's initial passes are discussed in a companion paper .
The electron precipitation responsible for Jupiter's auroral oval resembles the discrete and wave-induced aurora at Earth. The causative downward electron flux carries upward-directed magnetic field-aligned currents (FACs), largely generated by the breakdown of plasma corotation on closed magnetic field lines extending (beyond ~20 R J) (13, 14), a consequence of the fast rotation of the ...
We find that for the head-on collision, a post-impact central temperature of around 30,000 K leads to a present-day Jupiter with a diluted core. If the initial temperature profile is shaped by the ...
Jupiter's soft x-ray aurorae are produced by energetic [~megaelectron volts (MeV)] heavy ions (sulfur and oxygen), originally from the moon Io's volcanic activities ( 1 - 3 ). The dynamic x-ray emissions often pulse with a regular beat of a few tens of minutes ( 4, 5 ). The spectacular quasi-periodic auroral pulsations at Jupiter have ...
James Webb Space Telescope observations of Jupiter in July 2022 show these hazes in higher detail than ever before and reveal the presence of an intense (140 m s−1) equatorial jet at 100-200 ...
Read the paper: Jupiter's atmospheric jet streams extend thousands of kilometres deep. ... Research Highlight 19 JUL 24. NASA cancels $450-million mission to drill for ice on the Moon ...
Jupiter's density profiles as inferred by various structure models are shown in Fig. 1.Presented are profiles from Wahl et al. (2017) with a dilute core, a profile from Debras and Chabrier (2018) where Jupiter is found to have a large inner region with composition gradients, the density profile inferred by Vazan et al. (2018) from an evolution model that fit Jupiter's current properties (but ...
investigation of Jupiter's interior structure imply that the planet has compositional gradients and is accordingly non-adiabatic, with a complex internal structure. These new results imply that current models of Jupiter's formation and evolution require a revision. In this paper, we discuss
The Wide Field Camera 3 (WFC3) aboard the Hubble Space Telescope (HST) collected Jupiter images from 236 to 925 nm in 14 filters. The Near-Infrared Imager (NIRI) at Gemini North imaged Jovian thermal emission using a lucky-imaging approach (co-adding the sharpest frames taken from a sequence of short exposures), using the M ' filter at 4.7 μ m.
1 Introduction. Understanding how giant planets like Jupiter and Saturn form is a longstanding fundamental problem in planetary science. Until recently, nearly all of our understanding of this question has been drawn from theoretical considerations, observations of the compositions and structures of giant planets, and astronomical observations of protoplanetary disks.
The research paper, titled "How Jupiter's unusual magnetospheric topology structures its aurora," was written by Binzheng Zhang of the Department of Earth Sciences at the University of Hong Kong ...
If Jupiter was a hollow shell, 1,000 Earths could fit inside. Jupiter also is the oldest planet, forming from the dust and gases left over from the Sun's formation 4.5 billion years ago. But it has the shortest day in the solar system, taking only 10.5 hours to spin around once on its axis. Learn More about Jupiter
The Chinese scientific community is currently vigorously engaged in exploring the next frontier of Jovian system science and identifying the specific goals for the Chinese Jupiter mission through a series of workshops. This thematic collection will include papers presented at the Chinese Planetary Workshops for Jupiter Sciences.
The Juno mission flew through the plasma disk near the equator in Jupiter's magnetosphere frequently. We identify 274 plasma disk crossings of Juno between 10 and 40 R J from PJ5 to PJ44. Using a forward modeling method that combines the JADE-I time-of-flight and SPECIES data sets, we perform a survey of ion properties in the plasma disk.
The paper highlights important achievements in Jupiter study during the last few decades starting from early seventies. During the first two decades of its journey both 'Pioneer' and 'Voyager ...
Current information on the neutral atmosphere of Jupiter is reviewed, with approximately equal emphasis on composition and thermal structure on the one hand, and markings and dynamics on the other. Studies based on Pioneer 10 and 11 data are used to refine the atmospheric model. Data on the interior are reviewed for the information they provide on the deep atmosphere. The markings and dynamics ...
The research paper, titled "How Jupiter's unusual magnetospheric topology structures its aurora," was written by Binzheng Zhang of the Department of Earth Sciences at the University of Hong Kong ...
Pioneer 10. NASA's first spacecraft to visit the outer planets, Pioneer 10 was designed as a 21-month mission to Jupiter, yet lasted more than 30 years. After its Jupiter encounter in 1973, it continued beyond the solar system, sending its last signal to Earth in January 2003 from a distance of 7.6 billion miles. Explore.
Jupiter's Great Red Spot — the biggest windstorm in the solar system — is shrinking, and a new study may help explain why. Located in Jupiter's southern hemisphere, the Great Red Spot is a swirling, red-orange oval of high pressure more than 10,000 miles wide. It consistently blows more than ...
Using the James Webb Space Telescope (JWST), an MPIA-led team of astronomers imaged a new exoplanet that orbits a star in the nearby triple system Epsilon Indi. The planet is a cold super-Jupiter ...
The determination of Jupiter's odd gravitational harmonics by the Juno spacecraft reveals that the observed jet streams extend to about three thousand kilometres below the cloud tops. The Juno ...
Jupiter as seen by the space probe Cassini. Flights from Earth to other planets in the Solar System have a high energy cost. It requires almost the same amount of energy for a spacecraft to reach Jupiter from Earth's orbit as it does to lift it into orbit in the first place. In astrodynamics, this energy expenditure is defined by the net change in the spacecraft's velocity, or delta-v.
During its 61st close flyby of Jupiter on May 12, 2024, NASA's Juno spacecraft captured this color-enhanced view of the giant planet's northern hemisphere. It provides a detailed view of chaotic clouds and cyclonic storms in an area known to scientists as a folded filamentary region.
Those measurements confirmed that a Jupiter-sized planet was orbiting the star and that its orbit was highly eccentric, bringing the planet extremely close to the star before flinging it far out. Prior to this detection, astronomers had known of only one other planet, HD 80606 b, that was thought to be an early hot Jupiter.
The launch of NASA's James Webb Space Telescope (JWST) in 2021 kicked off an exciting new era for exoplanet research, especially for scientists looking at terrestrial planets orbiting stars other than our sun. But three years into the telescope's mission, some scientists have run into challenges that have slowed down progress.
Images of nearest 'super-Jupiter' open a new window to exoplanet research. ScienceDaily . Retrieved July 25, 2024 from www.sciencedaily.com / releases / 2024 / 07 / 240724123002.htm
Turkiye's defence industry has undergone dramatic changes over the last 50 years and the country has become a significant defence exporter. In this report, as part of a joint project with the IISS, researchers from the Center for Foreign Policy and Peace Research explore this process and the issues lying ahead.
The launch of NASA's James Webb Space Telescope (JWST) in 2021 kicked off an exciting new era for exoplanet research, especially for scientists looking at terrestrial planets orbiting stars other ...
The spectroscopic and photometric observations of a high-mass, transiting warm Jupiter, TIC 241249530 b, with an orbital eccentricity of 0.94, provide evidence that hot Jupiters may have formed by ...
De Freitas, Julian, Ahmet K Uguralp, Zeliha O Uguralp, and Puntoni Stefano. "AI Companions Reduce Loneliness." Harvard Business School Working Paper, No. 24-078, June 2024 ...