Advertisement

Analysis of the 2020 Taal Volcano tephra fall deposits from crowdsourced information and field data
- Research Article
- Open access
- Published: 02 March 2022
- Volume 84 , article number 35 , ( 2022 )
Cite this article
You have full access to this open access article
- M. I. R. Balangue-Tarriela 1 ,
- A. M. F. Lagmay ORCID: orcid.org/0000-0001-9672-9389 1 , 2 ,
- D. M. Sarmiento 1 ,
- J. Vasquez 2 ,
- M. C. Baldago 1 ,
- R. Ybañez 1 ,
- A. A. Ybañez 2 ,
- J. R. Trinidad 1 ,
- S. Thivet 3 ,
- L. Gurioli 3 ,
- B. Van Wyk de Vries 3 ,
- M. Aurelio 1 ,
- D. J. Rafael 1 ,
- A. Bermas 2 &
- J. A. Escudero 1
13k Accesses
6 Citations
7 Altmetric
Explore all metrics
After 43 years of dormancy, Taal Volcano violently erupted in January 2020 forming a towering eruption plume. The fall deposits covered an area of 8605 km 2 , which includes Metro Manila of the National Capital Region of the Philippines. The tephra fall caused damage to crops, traffic congestion, roof collapse, and changes in air quality in the affected areas. In a tropical region where heavy rains are frequent, immediate collection of data is crucial in order to preserve the tephra fall deposit record, which is readily washed away by surface water runoff and prevailing winds. Crowdsourcing, field surveys, and laboratory analysis of the tephra fall deposits were conducted to document and characterize the tephra fall deposits of the 2020 Taal Volcano eruption and their impacts. Results show that the tephra fall deposit thins downwind exponentially with a thickness half distance of about 1.40 km and 9.49 km for the proximal and distal exponential segments, respectively. The total calculated volume of erupted fallout deposit is 0.057 km 3 , 0.042 km 3 , or 0.090 km 3 using the exponential, power-law, and Weibull models, respectively, and all translate to a VEI of 3. However, using a probabilistic approach (Weibull method) with 90% confidence interval, the volume estimate is as high as 0.097 km 3 . With the addition of the base surge deposits amounting to 0.019 km 3 , the volume translates to a VEI of 4, consistent with the classification for the observed height and umbrella radius of the 2020 main eruption plume. VEI 4 is also consistent with the calculated median eruption plume height of 17.8 km and sub-plinian classification based on combined analysis of isopleth and isopach data. Phreatomagmatic activity originated from a vent located in Taal Volcano’s Main Crater Lake (MCL), which contained 42 million m 3 of water. This eruptive style is further supported by the characteristics of the ash grain components of the distal 12 January 2020 tephra fall deposits, consisting dominantly of andesitic vitric fragments (83–90%). Other components of the fall deposits are lithic (7–11%) and crystal (less than 6%) grains. Further textural and geochemical analysis of these tephra fall deposits contributes to better understand the volcanic processes that occurred at Taal Volcano, one of the 16 Decade Volcanoes identified by the International Association of Volcanology and Chemistry of the Earth’s Interior (IAVCEI) because of its destructive nature and proximity to densely populated areas. The crowdsourcing initiative provided a significant portion of the data used for this study while at the same time educating and empowering the community to build resilience.
Similar content being viewed by others
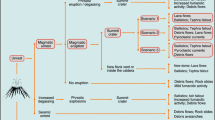
Probabilistic volcanic hazard assessment at an active but under-monitored volcano: Ceboruco, Mexico
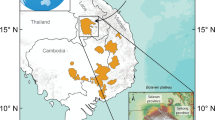
Assessing volcanic hazard and exposure to lava flows at remote volcanic fields: a case study from the Bolaven Volcanic Field, Laos
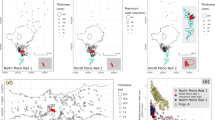
A new method to identify the source vent location of tephra fall deposits: development, testing, and application to key Quaternary eruptions of Western North America
Avoid common mistakes on your manuscript.
Introduction
After 43 years of repose, Taal Volcano violently erupted on 12 January 2020, forming a plume that reached 17–21 km above sea level (PTCC 2020 ; Perttu et al. 2020 ; Bachmeier 2020 ). The eruption started at around 1 p.m. (all times described in this manuscript are local times, which correspond to UTC+ 8) as a series of phreatic explosions involving heated ground water or lake water. The volcano Alert Level was raised to 2 at 2:30 p.m. because of the escalating volcanic activity. It was raised further on the same day to Alert Level 3 by 4:00 p.m. and then to Alert Level 4 by 7:30 p.m. Alert Level 5, the highest category on a scale of 0–5, which means that a hazardous eruption is ongoing, was not raised. According to the Philippine Institute of Volcanology and Seismology (PHIVOLCS), the agency mandated to mitigate disasters that may arise from volcanic eruptions, progression from phreatic to a phreatomagmatic eruption took place at about 5:00 p.m., when magma came into contact with groundwater or lake water from the Main Crater Lake (MCL) of Taal Volcano Island (TVI) (PHIVOLCS 2020a ). The tall volcanic plume and large associated umbrella cloud of the main eruption resulted in tephra (ash) fall to the north–northeast as far as the capital city of Manila, 65 km from the active vent. By 2:49 a.m. of the following day (13 January 2020), the activity transitioned to lava fountaining. The change in eruptive style was the start of waning activity, characterized by discrete cannon-like explosions that generated 2-km-high bent-over plumes during the rest of the second day and eruption columns of various heights less than 1 km that lasted until 22 January 2020. Steam-laden plumes persisted in the next few weeks with decreasing intensity.
Many local tourists and residents around Taal Lake witnessed the explosive eruption as the event happened on a weekend. As news of the eruption spread, some authors of this paper rushed towards TVI to document the event through photographs and videos, and by sampling tephra for further analysis. The lead authors from the University of the Philippines (UP) have research and public service mandates (Congress of the Philippines 2020 ). Some are also researchers of the UP Resilience Institute, whose mission is to empower local communities through multidisciplinary actions toward resilience. As such, an independent quick response team was formed to solicit crowdsourced information from the public through social media outlets. Residents affected by the tephra fall, known as ashfall to the layman, were requested through social media to document and collect samples before they were washed out by rain or swept away. In the weeks following the main eruption of Taal Volcano, the team conducted fieldwork to measure tephra fall thickness and collect samples in different locations where tephra falls were reported to have been deposited.
This paper describes the collective work done to document details of the eruption sequence and impacts of tephra fall of the 2020 Taal Volcano eruption. A significant amount of data comprising this paper is owed to the efforts of the general public who immediately reported their observations and contributed tephra samples around Taal Volcano. It was a unique and significant opportunity to engage the public in citizen science, with the crowdsourcing initiative providing the authors with invaluable data while raising awareness through engagement in the observation of volcanic hazards phenomena. As one of the 16 Decade Volcanoes identified by the International Association of Volcanology and Chemistry of the Earth’s Interior (IAVCEI), this work is of significant value, most notably in light of Taal Volcano’s destructive nature and proximity to densely populated areas (Torres et al. 1995 ), including the country’s National Capital Region, a metropolis inhabited by more than 13 million people (PSA 2021 ).
Methodology
To characterize Taal Volcano’s 2020 eruption through its tephra deposits, ground observations, satellite remote sensing, crowdsourcing, field validation, numerical simulations, and laboratory analysis were performed. Each of these methods are described below.
Ground and satellite observations
Photos taken by residents around Taal Lake, weekend tourists, and passengers of commercial airplanes were reviewed to determine the type of eruption that took place on 12–13 January. Most of these photographs are available from the Internet but some were provided directly to the authors. Time-lapse videos taken by the authors on 13 January from Tagaytay, north of TVI, were also reviewed.
The eruption plume was directly observed and photographed by some of the authors from a distance of about 22 km in the late afternoon of 12 January. The following day, the eruption was observed from the northern ridge of Taal Caldera. Time-lapse videos of the eruption were taken during the observation periods (see Supplementary Files ).
Himawari satellite images (Bachmeier 2020 ) were used to measure the umbrella cloud radius. These were compared with estimates of the eruption cloud height of the cataclysmic event on 12 January determined by the Pacific Tropical Cyclone Center (PTCC) (Perttu et al. 2020 ; PTCC 2020 ) and the Cooperative Institute for Meteorological Satellite Studies (CIMSS) (Bachmeier 2020 ) and used to determine the Volcanic Explosivity Index (VEI) (Constantinescu et al. 2021 ).
Crowdsourced accounts and field observations
Recognizing that the Philippines remains the leader in social media and Internet usage worldwide (Baclig 2020 ) and the fact that tephra fall deposits are easily removed by water erosion in tropical regions, an interactive Google Map was created on the morning of 13 January (Fig. 1 a) for “netizens,” or citizens who are highly active on social media and other online platforms, to quickly report the pin location and estimated thickness of ashfall deposits in their area. The interactive map also allowed attachment of photos to help visualize the ashfall deposit thickness. Social media posts on Facebook and Twitter containing reports of ashfall were integrated into the Google Map. The advantage of using Google Maps is that contributors could visualize ashfall reports and how their individual pins shaped the data and the map.
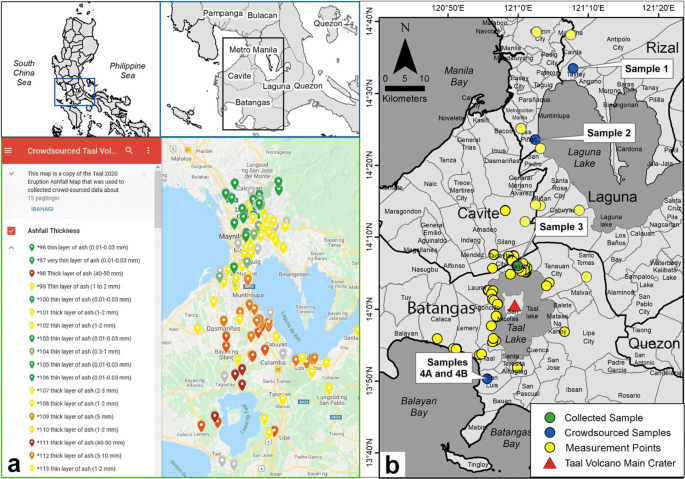
(a) Interactive Google Map showing the locations of ashfall occurrences as reported by netizens. Each pin corresponds to an ashfall thickness in that site: dark green, 0.01 to 0.03 mm; light green, 0.30 to 1.00 mm; yellow, 1.00 to 3.00 mm; orange, 3 to 10 mm; light red, 10 to 30 mm; and dark red, 30–100 mm. No report for ashfall with thickness of 0.03–0.30 mm was received through the Google Map platform. (b) Map showing the location of measurement and sampling points during the field validation
The use of hashtags such as #TaalVolcano, #iTalaAngTaalAsh (translation: #RecordTheTaalAsh), and #TaalAshfall were particularly useful in consolidating the posts, tweets, and news regarding the event. Informative discussions on the on-going state of Taal Volcano and its implications were formed in Twitter and Facebook, engaging everyone to participate and spread awareness. In addition, the hashtags were used to pinpoint the location of severely affected areas needing donations, relief efforts, and rescue.
The authors also focused on public communication, leading forums, and producing infographics about the eruption. The discussions on the original posts in social media lasted for over a week on Twitter and Facebook. The calls for crowdsourced data were retweeted by a media outfit, a science organization (AGHAM, Advocates of Science and Technology for the People) as well as local and international scientists with one post garnering a total of 372,003 impressions, 86,206 engagements, 1954 likes, and 1436 retweets. The interactive Google Map reached over 3000 views and received 247 unique submissions.
All reports were vetted to assess the quality of thickness measurements and only those with associated photographs were considered. Of the 247 reports, 167 thickness measurements were deemed accurate. Contour lines were drawn on points of similar tephra fall deposit thicknesses to construct a GIS isopach map.
A series of field surveys were conducted by the Quick Response Team of the UP National Institute of Geological Sciences and UP Resilience Institute in the following municipalities: (1) Agoncillo, Batangas; (2) Calaca, Batangas; (3) Laurel, Batangas; (4) Lemery, Batangas; (5) San Nicolas, Batangas; (6) Taal, Batangas; (7) Talisay, Batangas; (8) Tanauan, Batangas; (9) Silang, Cavite; and (10) Tagaytay City, Cavite. Observation and measurement points were chosen to show optimal preservation of the deposits (Fig. 1 b). Tephra fall thicknesses were most reliable when measured on house and car rooftops, bridge columns and railings, road and fence ledges, fallen trees, and tombs in cemeteries. The total lockdown in some places due to the eruption significantly reduced anthropogenic activity, reducing reworking of the tephra and preserved the tephra fall deposits in many areas for weeks. Tephra samples were collected and stored in resealable plastic bags with several samples solicited from colleagues residing in towns where tephra fall occurred.
Eruption parameter calculations
TephraFits was used to model the Taal Volcano’s 2020 main eruptive episode based on the crowdsourced and surveyed tephra fall data. It is a Matlab function, a collection of codes dedicated to the characterization of tephra fall deposits such as AshCalc (Daggitt et al. 2014 ) and TError (Biass et al. 2014 ). The TephraFits program enables the user to quickly compute eruption parameters including the deposit volume and mass, VEI, magnitude, and rates of thickness-decay away from the source and allows for better eruption classification using deposit-based schemes (Biass et al. 2019 ). Outputs generated by the TephraFits algorithm follow three well-known and frequently used fitting methods, which are the exponential, power-law, and Weibull integration models.
Exponential model
The exponential model is an integration strategy that describes the relationship between the deposit thickness, T , as a function of the root isopach area, x as follows (Eq. 1 ):
where c represents the theoretical maximum thickness located at the vent and m describes the rate of decrease in tephra deposit thickness (Pyle 1989 ; Biass et al. 2019 ). This equation is linearized by taking the logarithm of both sides of the equation and least squares regression is applied to get the values for parameters, c and m (Daggitt et al. 2014 ).
Multiple exponential segments are applied to the data so that the exponential law allows for modelling deposits of varying thinning rates with distance away from the vent as displayed by many well-constrained tephra fall deposits (Pyle 1989 ; 1990 ; Fierstein and Nathenson 1992 ; Bonadonna and Houghton 2005 ; Watt et al. 2009 ). This integration approach is effective for simple approximations such as determining the minimum volume of a deposit given sparse data points (e.g., Pyle 1995 ; 1999 ; Legros 2000 ; Sulpizio 2005 ) (Daggitt et al. 2014 ).
Power-law model
The power-law model is another fitting method that describes the relation between thickness, T , as a function of root isopach area, x , as follows (Eq. 2 ):
where c represents a linear scaling factor and m , characterizes the rate of decrease in tephra deposit thickness (Bonadonna et al. 1998 ; Bonadonna and Houghton 2005 ). Similar to the exponential model, this equation is linearized by taking the logarithm of both sides of the equation and least squares regression is applied to get the values for parameters, c and m . The main disadvantage of using the power-law model is that T(x) is not integrable between 0 and \(\infty \) . Thus, the proximal and distal limits of integration have to be selected (e.g., Bonadonna and Costa 2012 ) (Daggitt et al. 2014 ).
Weibull model
Of the three fitting methods, the Weibull model was proposed more recently as it combines the advantage of the exponential model being integrable between 0 and \(\infty \) and the power-law model’s use of variable rates of decrease in deposit thickness (Bonadonna and Costa 2012 ). The model describes the relationship between thickness, T , and root isopach area, x , as follows (Eq. 2 ):
The additional parameter, λ , allows the Weibull model to capture variation in the deposit’s thinning rate, which in the exponential model necessitates multiple segments (Daggitt et al. 2014 ).
For the exponential, power-law, and Weibull fitting, the implementation strategies recommended by Biass et al. ( 2019 ) were used in this study. A 10% error was applied to all thickness and diameter measurements and a 20% error on the distal limit ( C ). Several iterative runs were conducted while observing strict statistical measures and ensuring critical interpretation that would match geologically realistic values. For example, the 𝜃 output for the Weibull Fit was ensured to agree with the thickness ( \(\sim \) 1 m) and maximum grain size values ( \(\sim \) 11 cm) of tephra fall deposits observed near the crater rim where there were no identified base surge deposits (Lagmay et al. 2021 ). Values were compared to tephra fall deposits from other volcanoes in the global dataset to ensure they were realistic (Bonadonna and Costa 2013 ; Daggitt et al. 2014 ; Biass et al. 2019 ). Further sensitivity analyses were performed for output values, especially for the distal limits where thickness values are harder to constrain (sub mm) and preservation is short-lived. A list of the final selected input parameters used for the results presented in this work is shown in Table 1 .
Furthermore, this study used the Weibull method to estimate the plume height according to equation 7 of Bonadonna and Costa ( 2013 ). The modeled eruption height values were then compared with those measured from satellite data (Perttu et al. 2020 ; Bachmeier 2020 ). Magnitude-related parameters such as thickness half-distance, b t and λ T H , as well as intensity-related parameters, in particular thickness half-clast, b c and λ M L (Biass et al. 2014 ), were used to classify the 2020 Taal eruption according to the schemes of Pyle ( 1989 ) and Bonadonna and Costa ( 2013 ).
Limitations
Circumspection on the use of TephraFits is hereby noted. Our calculations are based on crowdsourced and field survey data, which are relatively scarce point observations of the entire tephra fall deposit. More thickness and maximum grain-size measurements could have been added, but lockdown restrictions due to the raised alert level for Taal Volcano and later for COVID-19 prevented further collection of data. Most of the crowdsourced data were visually estimated from the photos submitted whereas field surveys were delayed by 1–2 weeks. Erosion by wind and surface water runoff may have reduced the thickness of the fall deposits. Furthermore, the data points were subjectively contoured to generate isopach and isopleth maps used to constrain transport and depositional processes. As such, many sources of uncertainties interacted and propagated to the final results presented in this work (Biass et al. 2019 ). Nonetheless, these caveats guided us in the critical use and interpretation of the models, ensuring that they reflect geological reality and direct observations of Taal Volcano’s main eruption.
Duration of peak activity
The duration of peak activity was determined from the review of proximal seismic data recorded by a station north of TVI in Dasmarinas, Cavite. Data were retrieved from the International Federation of Digital Seismograph Networks (FDSN) (Fig. 2 ), which provides open access to seismic data from the Philippines. The seismic records came from the nearest station to Taal, which is part of a growing network of low-cost but professional seismometers (Anthony et al. 2018 ; Bent et al. 2018 ; Holmgren and Werner 2021 ; Subedi et al. 2020 ) in the country, owned and operated by private entities and citizen scientists (Aurelio et al. 2020a ; 2020b ). The estimate of the duration of peak activity was compared with the accounts of eruptive activity in official bulletins of PHIVOLCS (Table 2 ), reports from the Global Volcanism Program, news articles, social media posts, and airlines and ground observations by the authors.
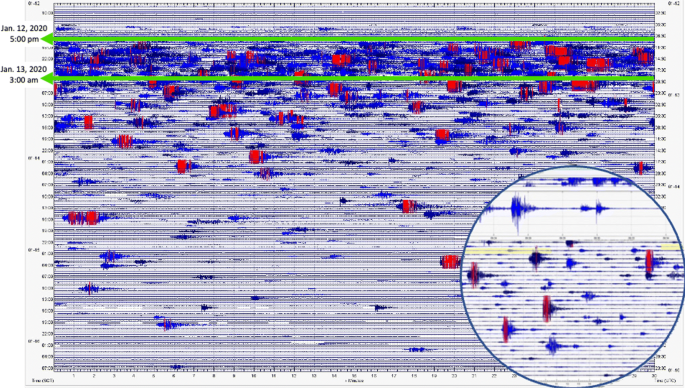
Seismogram from a public seismic network (PSN) station in Dasmarinas, Cavite, available from FDSN. Blue lines are seismic waveforms whereas the red lines are clipped waveforms due to their high amplitude. The estimated period of intense seismic activity corresponding to the development of the 17–21-km-high plume is approximately from 5 p.m. of 12 January to 3 a.m. the following day. The inset circle is the wave panel showing a magnified view of the recorded waveform with amplitude represented in counts
Laboratory analysis
Samples used for grain-size analysis conducted at the UP National Institute of Geological Sciences (UP NIGS) came from those collected during fieldwork and those solicited from colleagues (see Fig. 1 b for locations). For grain size analysis, the samples were oven dried for 6–8 h and reduced to 100 g using coning and quartering to eliminate systematic biases. The maximum grain sizes of predominantly equant tephra grains utilized in the isopleth maps came from twenty samples that were handpicked and measured using a micrometer. Manual sieving was then carried out on three samples using the No. 18 (1.00 mm, 0 ϕ ), No. 35 (0.50 mm, 1 ϕ ), No. 60 (0.25 mm, 2 ϕ ), No. 120 (0.125 mm, 3 ϕ ), and No. 230 (0.063 mm, 4 ϕ ) test sieves of the U.S.A. Standard Test Sieves ASTM E-11-20 (International ASTM 2014 ) to determine the size frequency distribution. Using an analytical balance, size fractions were weighed, and those data were plotted on a standard grain-size distribution graph. The manually sieved tephra samples were then sonicated to separate aggregates for componentry analysis using a Zeiss stereomicroscope. The relative abundance of each component was assessed by grain counting at least 300 particles per size fraction (> 125–250 μm, 250–500 μm, 500 μm–1 mm, 1–2 mm, 2–4 mm, and > 4 mm) for each sample.
Two additional samples were sent to the Laboratoire Magmas et Volcans, University Clermont Auvergne (LMV-UCA), for further analyses on size frequency distribution, texture, morphology, and geochemistry. Both samples were collected at the same location 20 km from the eruptive vent (sample 4A and sample 4B) on 13 January (see Fig. 1 b for location). The first (sample 4A) was collected at 10:22 a.m. and the second (sample 4B) at 1:05 p.m. Grain sizes for both samples were first measured by manual sieving following the same procedure as described above. Particle size distribution (PSD) was measured on size fractions below 1 mm in diameter using a MALVERN Mastersizer 300 laser diffractometer, following the Thivet et al. ( 2020 ) procedure.
The following textural and chemical analyses were then performed on the sample grain-size modes (125–90 μm). Componentry was determined on the 2 samples from epoxy-impregnated polished sections using a JEOL JSM-5910 LV scanning electron microscope (SEM). The 2D internal textures of the particles were investigated with backscattered electron (BSE) imagery with an acceleration voltage of 15 kV. At least 100 particles were counted for each sample. The 3D particle surfaces were imaged using a CARL ZEISS Supra 55/55 VP field emission (FE) SEM and using secondary electron (SE) imagery, with an acceleration voltage of 3 kV.
Finally, 2D ash morphology was quantified using a MALVERN Morphologi G3 morpho-grainsizer following the Thivet et al. ( 2020 ) procedure. Measurements were performed on grain size modes of both samples. At least 1000 particles were analyzed for each sample and particle shapes were automatically measured by the instrument. The different shape parameters used in our study are described in Thivet et al. ( 2020 ). In our study, we represented particle morphology through two distinct roughness parameters, solidity (SLD) and convexity (CVX), which represent the morphological (particle scale) and the textural scale (smaller scale) roughness of the particles, respectively. A perfectly round or square particle has a SLD and CVX value of 1. On the other hand, SLD and CVX decrease as soon as shape irregularities appear.
In situ glass compositions were determined from microlite-free juvenile particles, using a Cameca SxFiveTactis electron probe micro-analyzer (EPMA), with an acceleration voltage of 15 kV, current intensity of 8 nA, and a laser beam of 10 microns, following the Gurioli et al. ( 2018 ) procedure.
Crowdsourced accounts
The majority of crowdsourced reports came from Metro Manila with fewer netizen reports received from the CALABARZON (Cavite, Laguna, Batangas, Rizal, and Quezon) Region. This can be attributed to the difference in population density between the National Capital Region and these adjacent provinces, and that most of CALABARZON was heavily affected by the eruption (Fig. 1 ). All residents within 14 km of TVI were ordered to evacuate and many other residents outside of this area voluntarily relocated to safety. Partial power interruptions in the municipalities of Cavite, Laguna, and Batangas lasting for days after the eruption (Del Castillo et al. 2020 ) likely contributed to fewer ashfall reports from these locations. Despite the smaller number of data reported from proximal locations, we consider the dataset robust enough when augmented with additional field observations.
The thinnest tephra fall deposits were reported north of Metropolitan Manila as well as in the provinces of Rizal, Bulacan, and Pampanga. These deposits were observed as dispersed tephra particles with a thickness of < 1 mm that were hardly visually discernible, particularly in urban to suburban areas.
Thicker deposits, with thickness of ≥ 1 mm, were more apparent and easily documented by netizens. These could be measured with a standard ruler or better estimated in photographs, and were reported in southern Metropolitan Manila and some portions of the provinces of Rizal, Cavite, and Laguna. Tephra fall deposits progressively thicken towards the eruption source. Deposits with thicknesses of at least 1 up to 10 mm (Fig. 3 c, d) fully blanketed the surface of roads, roofs, car hoods, and leaves of plants.
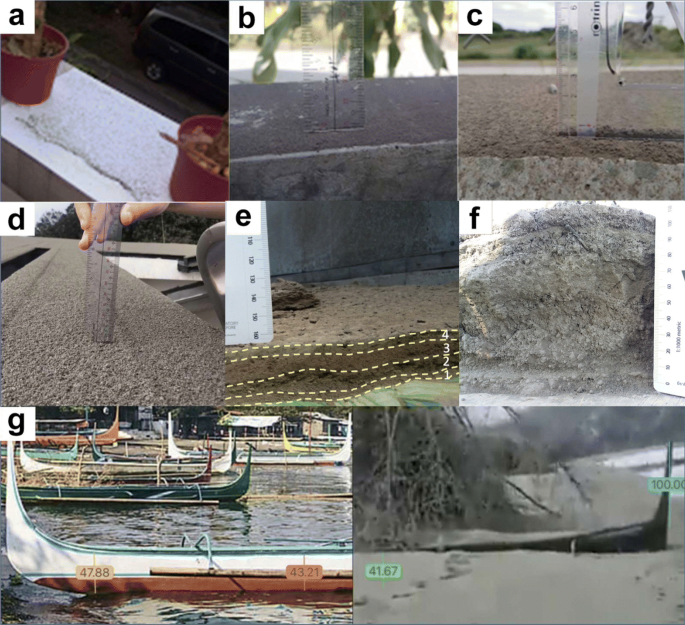
Representative photos of tephra fall deposits with varying thicknesses crowdsourced from netizens and from our fieldwork: (a) < 1 mm in Quezon City; (b) 1 mm in Quezon City; (c) 2.5 mm in Lipa City, Batangas; (d) 5 mm at Westgrove Heights, Silang, Cavite; (e) 40 mm in Talisay, Batangas (dashed lines represent different layers in the deposit); and (f) 110 mm in Agoncillo, Batangas; (g) 400–500 mm at the north shore of TVI. The two photos are of a banca, a small boat, typically used in the island. One photo was taken before the eruption; the other is a video screenshot from Facebook posted by GO Batangas on January 13. Numerical values in the photos refer to heights (in centimeters)
Tephra fall deposits closer to the source in Cavite, Laguna, and Batangas were much thicker with thicknesses of 10 to 30 mm (Fig. 3 e, f). Objects and structures situated outdoors were heavily blanketed with tephra. Public roads were also fully covered with thick tephra that required clearing for safe use. Netizens from these areas reported “smoking” ash outside of their homes as wind remobilized and dispersed tephra particles.
The thickest tephra fall deposits were reported in the municipalities and cities of Batangas immediately surrounding Taal Lake. These include Agoncillo, Calaca, Laurel, Lemery, Lipa, San Nicolas, Talisay, Taal, and Tanauan. Deposits 40 to 50 mm thick heavily damaged vegetation (Fig. 3 ). No netizen reports of ashfall from TVI were received.
Field observations
On 12 January, tephra from the eruption reaching plume heights of 17–21 km was first deposited southwest of Taal Volcano (Lagmay et al. 2021 ). A few hours later, the plume shifted north–northeastwards and wet tephra fall showered cities and municipalities 70 km north–northeast of the main crater. On 13 January, continuous activity at the volcano generated plume heights up to 2 km above sea level and an eruption cloud that drifted to the southwest of the volcano.
The tephra fall deposits exhibited parallel layerings made distinct by changes in color and grain size. In proximal areas, layers with ash-sized (< 2 mm in diameter) to lapilli-sized (> 2 mm diameter) lithic, vitric, and crystal fragments are observed in the lower layers. North of the volcano island, in Silang, Tagaytay, Talisay, and Tanauan, deposits have thicknesses from 1 to 4 cm with at least four layers. In Talisay, Batangas, 9 km from the crater, a deposit was observed to exhibit a light gray bottom layer (layer 1) overlain by a dark gray ash deposit (layer 2) with coarse accidental lithic lapilli, vitric grains, and free crystals (Fig. 3 e). The third layer (layer 3) is made up of brown ash and is the thickest. Finally, light gray ash makes up the topmost layer (layer 4). In Tagaytay, a similar deposit was observed but with the bottom-most light gray layer absent. Furthermore, most of the observed proximal deposits contained accretionary lapilli.
Deposit volume calculation
Thickness reports and field data reveal the approximate distribution of the tephra fall deposits of the 12–13 January eruption of Taal Volcano (Fig. 4 a). Ellipticity for all isopach areas range from 0.15 to 0.56, which implies elongated tephra fall deposition rather than an axial symmetry. The ellipticity of the tephra deposit is attributed to winds blowing toward the north–northeast at higher levels in the atmosphere during the main part of the eruption (i.e., 1:00 p.m. 12 January to 4:28 a.m. 13 January).
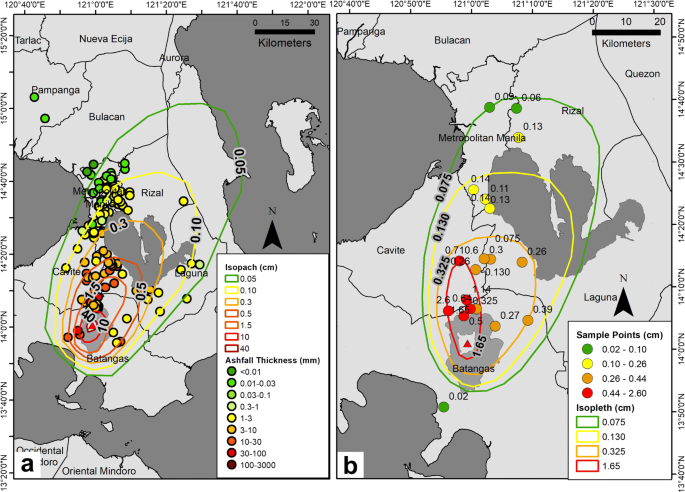
Isopach (a) and isopleth (b) maps generated from vetted crowdsourced accounts and field data corresponding to the 12 January to early morning (about 4:00 a.m.) of 13 January eruption of Taal Volcano
A total isopach map area of 8605 km 2 was covered by tephra fall after the 12 January eruption based on 0.05-cm depth, crowdsourced data, and field observation. Within this area, seven isopach contours were drawn (Fig. 4 a) corresponding to the reported thickness ranges of tephra fall after the eruption.
The isopleth contours were delineated based on the maximum grain sizes measured from the samples (Fig. 4 b) gathered during field validation. A gradual decrease of tephra grain sizes from the TVI to the surrounding communities can be observed on the map, with the smallest (0.02–0.10 cm) grain sizes sampled just within Metro Manila. The trend of decreasing tephra grain size also followed the north–northeast wind direction at the time.
The thickness and maximum grain size values used for the isopach and isopleth contours, respectively, are listed in Table 3 with their corresponding area in square kilometers. The thickest isopach contour, 40 mm, approximately encloses TVI and has a distance, r , of approximately 2 km away from the main crater. At this distance, the thickness of the tephra deposit was determined from the nearly complete burial of the hull of a banca, a traditional boat used in Taal Lake (Fig. 3 g). The thinnest measurement is ≤ 1 mm, reported by netizens in Quezon City in Metro Manila and in the Rizal/Quezon Province boundary.
The isopach contours show exponential thinning of tephra downwind with a 1.40-km thickness half-distance for the proximal segment and 9.49-km half-distance for the distal segment. Along the crosswind direction, the tephra fall deposit also thins exponentially with a 1.28-km thickness half-distance for the proximal segment and 1.50-km half-distance for the distal segment (Fig. 5 ).
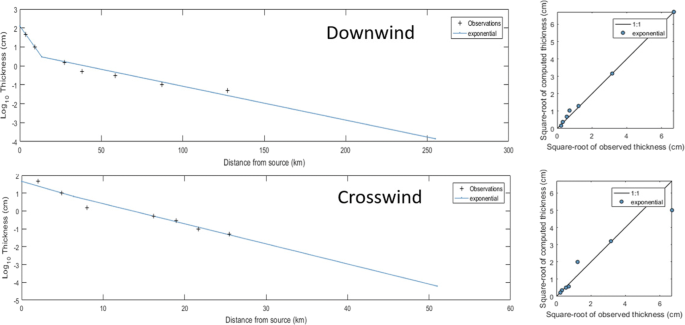
Thickness half-distance exponential plot of the downwind and crosswind segments for Taal Volcano’s tephra fall deposits
Results on the volume of Taal Volcano’s 2020 tephra fall deposit differ based on the model used (Fig. 6 ). The exponential model yields a volume of 0.057 km 3 , whereas the power-law model calculates a value of 0.042 km 3 . The Weibull model gives the largest volume of 0.090 km 3 . All are within the volume range of 0.01–0.1 km 3 , which translates to a VEI of 3.
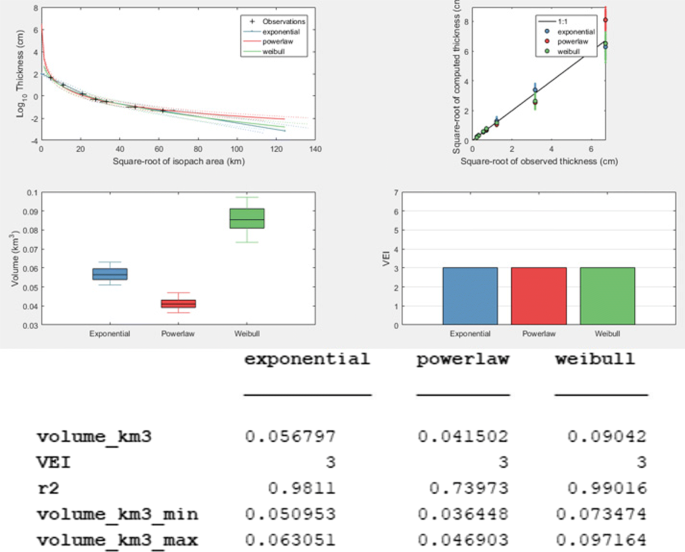
Results of the TephraFits model calculations using the exponential, power-law, and Weibull methods
Using the probabilistic approach with 90% confidence intervals or the 5–95th percentile intervals, the range of volumes become 0.051–0.063 km 3 (exponential), 0.036–0.047 km 3 (power-law), and 0.073–0.097 km 3 (Weibull), which shows that the Weibull fit is sensitive to uncertainties regarding isopach thickness and the square root of the isopach area. In the Weibull fit, the 95th percentile of the distribution results in a volume of 0.097 km 3 , which gets very close to the volume that corresponds to VEI 4 (0.1–1 km 3 ).
TephraFits calculations using the Weibull method based on isopleth data show a median eruption height of 17.8 km above vent. Considering typical uncertainties of 20 % for determining plume heights (Bonadonna and Costa 2013 ), the range of plume heights determined for the main eruption of Taal Volcano is 14.2–21.3 km above vent. In terms of the classification of the eruption, we calculate λ T H and \(\frac {\lambda _{MC}}{\lambda _{TH}}\) , as well as thickness half-distance b t and thickness half-distance over thickness half-clast ( \(\frac {b_{t}}{b_{c}}\) ) values that plot in the sub-plinian fields of Pyle ( 1989 ) and Bonadonna and Costa ( 2013 ) (Fig. 7 ).
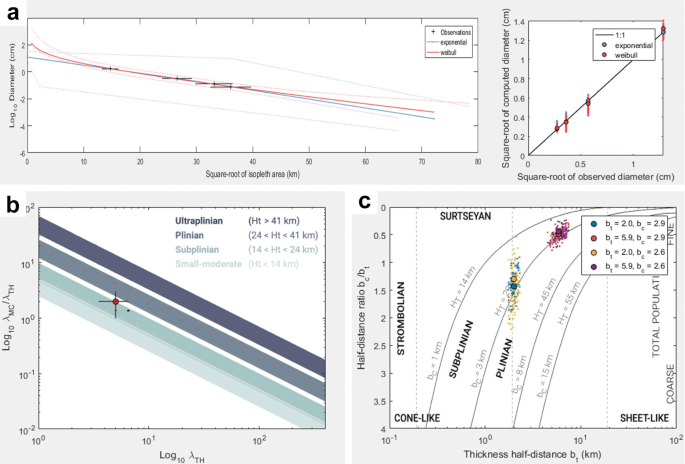
Results of the TephraFits calculations for isopleth data (a) and the classification schemes according to Bonadonna and Costa ( 2013 ) (b) and Pyle ( 1989 ) (c). Both plots in the sub-plinian field
Tephra sample characterization
Grain-size distributions of the tephra fall deposits from samples collected north–northeast of Taal Volcano show a unimodal distribution (Fig. 8 a). The grain-size frequency plot for the most proximal deposits shows an asymmetrical distribution, skewed towards the finer grain sizes. Coarser tephra grains were absent for the two distal deposits collected in Muntinlupa, Metro Manila, and Taytay, Rizal, with grain-size frequency plots also displaying a single mode at 125–500 μm.
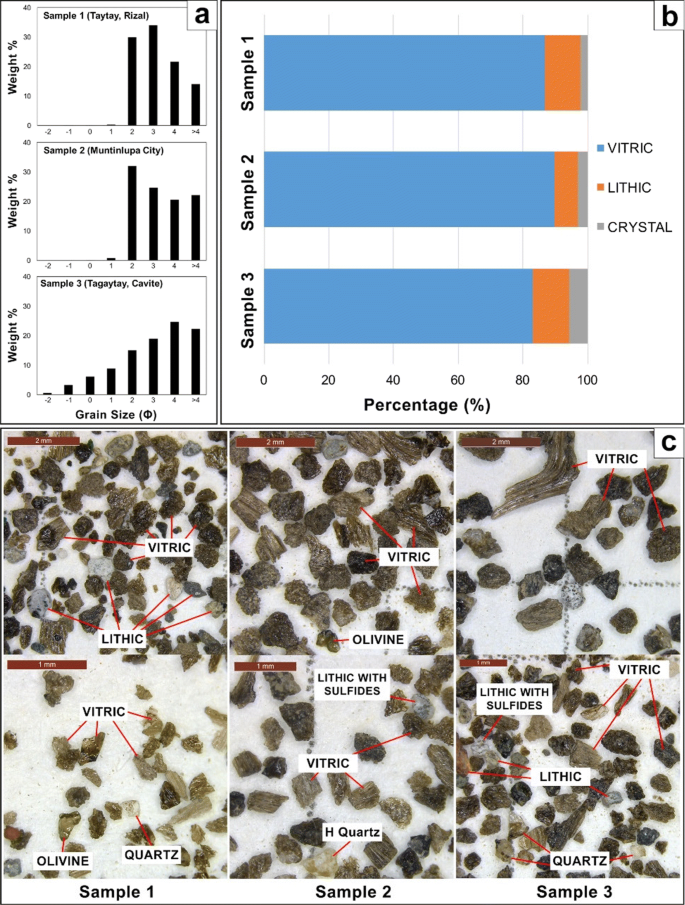
Samples 1, 2, and 3 analyzed at UP-NIGS. (a) Grain-size distribution for the tephra fall deposit in different localities. (b) Relative percentage of the tephra components. (c) Tephra components including vitric, lithic, and crystals (olivine, quartz), as well as secondary quartz (H quartz) and sulfides adhering to the rock fragments
Grain-size distributions of the samples collected south–southwest of Taal Volcano (Fig. 9 a) range between 300 μm (1.75 ϕ ) and 1 μm (10 ϕ ) and show a single mode at 125–190 μm (3–3.5 ϕ ). These volume distributions (which reflect mass distributions assuming a constant particle density for each grain size bin) are asymmetric and skewed toward relatively coarse grain sizes. Note that both bulk samples contained aggregates that were crushed during the analysis. These aggregates were irregular in shape with no specific internal grain-size organization.
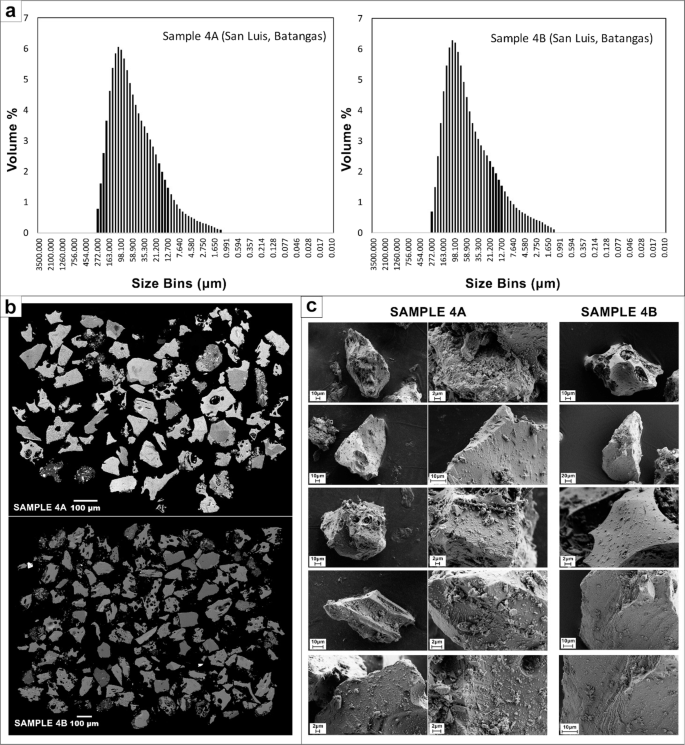
Samples 4A and 4B analyzed at LMV-UCA. (a) Grain-size distribution from laser diffraction measurements, (b) BSE images, (c) SE images
Binocular microscope analysis showed that the 2020 Taal Volcano tephra consists of volcanic glass (vitric), accidental lithic fragments, crystals, and crystal fragments (Fig. 8 c). Grain counting reveals that volcanic glass is the dominant component in all the samples. Volcanic glass or the vitric components consist of about 83–90%. Lithic grains only represent 7–11%, whereas crystal fragments compose less than 6% of the tephra material (Fig. 8 b).
Vitric components are translucent light brown to black. The grains vary from blocky to fluted to scoriaceous forms. Lithic fragments, on the other hand, include whitish yellow hydrothermally altered fragments, sub-rounded volcanic rock fragments, and reddish-orange oxidized grains. Crystal fragments of olivine, quartz, and plagioclase were observed as free crystals embedded within glass. Sulfides were also observed in some of the hydrothermal fragments (Fig. 8 c).
Backscatter electron (BSE) image analysis of each of the grain size modes of samples 4A and 4B (Fig. 9 b) was used to identify different components, including juvenile glassy (sideromelane) particles (80%), juvenile microlite-rich (tachylite) particles (10%), scarce phenocrysts of olivine and plagioclase (10%), and very scarce non-juvenile hydrothermal fragments (< 1%).
Scanning electron (SE) images (Fig. 9 c) confirmed the presence of abundant fine adhering particles. The surfaces of juvenile glassy particles are typically composed of stepped features and microfractures.
Tephra morphology measurements (Fig. 10 ) reflect the coexistence of both irregular (glassy and transparent textures) and blocky (microlite-rich and opaque textures) juvenile particles, as well as blocky crystals (conchoidal fractures and transparent textures).
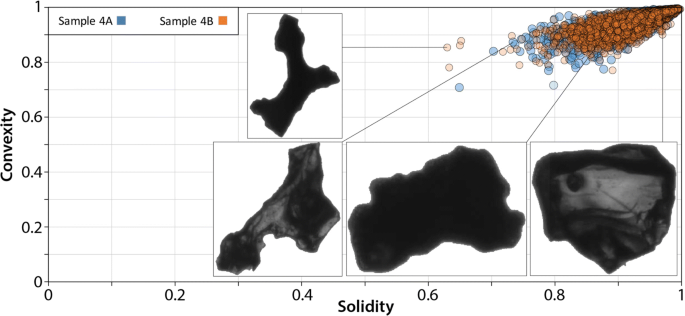
Tephra morphology measurements, representing convexity vs. solidity parameters. Analysis conducted at LMV-UCA
In situ glass compositions measured on juvenile microlite-free tephra particles reveal that the erupted magma is andesitic in composition, with no significant variations within the two samples from Taal Volcano’s 12 January to early morning of 13 January eruption (Fig. 11 ).
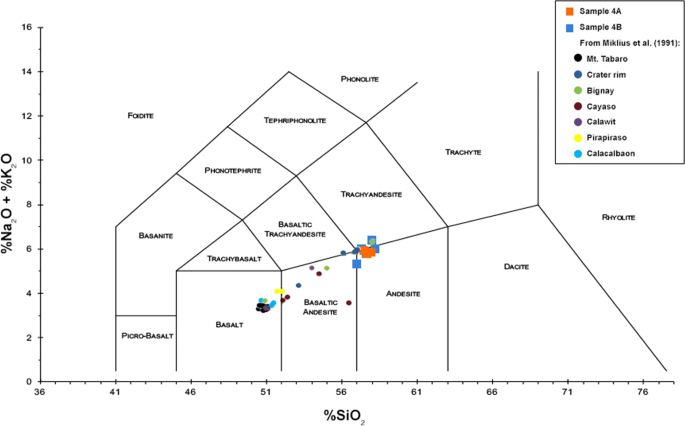
Plot of the geochemical analysis in this work against the whole-rock geochemistry of lava flow deposits and loose rocks from previous eruptions of Taal Volcano (Miklius et al. 1991 ). In situ glass compositions measured on juvenile microlite-free particles show an alkali-rich andesitic composition in the TAS diagram
Analysis of isopach and isopleth data
The tephra fall of Taal Volcano during its 2020 unrest was dispersed over a large region covering an approximate area of at least 8605 km 2 . In a tropical environment where rainfall is common, tephra deposits can be easily washed away and deliberately removed by residents, forever erasing their depositional record. Using combined crowdsourcing the day after the eruption and field surveys within weeks after the event, tephra fall deposits were systematically documented, revealing some insights on the characteristics of the 12 January to early morning of 13 January eruption of Taal Volcano. Ashfall deposit measurement was found to be more accurate on flat, horizontal, and undisturbed surfaces. Field sampling focused on such areas and would make better observation points for netizens in future implementations of crowdsourcing ashfall data.
Using TephraFits to determine the thickness half-distance of tephra fall deposits, we calculate 1.40 km and 9.49 km for the proximal and distal exponential segments, respectively. The total calculated volume of erupted tephra for the 12 January to early morning of 13 January main eruption is 0.057 km 3 (exponential model), 0.042 km 3 (power-law model), and 0.090 km 3 (Weibull model). All these computed values translate to a VEI of 3.
However, using the probabilistic approach (Weibull method) with 90% confidence interval, the volume estimate can be as high as 0.097 km 3 which is close to the lower end of the volume range of VEI 4 (0.1–1 km 3 ). Adding the volume of the base surge deposits amounting to 0.019 km 3 (Lagmay et al. 2021 ), the total erupted volume translates to a VEI of 4, consistent with the classification criteria of Constantinescu et al. ( 2021 ), namely (1) volume of ejecta; (2) eruption plume height; and (3) umbrella cloud radius. The total erupted volume was calculated using crowdsourced and field survey data, whereas the erupted height and umbrella cloud radius are direct measurements from satellite data of Taal Volcano’s 2020 main eruption plume (Bachmeier 2020 ; Perttu et al. 2020 ; Lagmay et al. 2021 ).
Discrepancies between the power-law, exponential, and Weibull volumes which lead to different estimates of VEI values have been documented for other volcanoes. For example, in Layer 5 of the Cotopaxi (1180 ± 80 years B.P.) fall deposits, both power-law and exponential methods yielded a VEI 4 whereas the Weibull method resulted in a VEI 5 estimate (Biass et al. 2019 ). For Taal, all methods have a resulting volume corresponding to a VEI 3. A VEI 4 volume can be reached if the largest end of the largest estimate (Weibull method) is used with the addition of the base surge deposits. This is more consistent with the VEI classification of Constantinescu et al. ( 2021 ) for the observed plume height of 17–21 km (Bachmeier 2020 ; Perttu et al. 2020 ) and umbrella radius of 100 km (Lagmay et al. 2021 ) of Taal Volcano’s main eruption in 2020.
An eruption size at the low end of a VEI 4 perhaps explains the smaller volume and limited distribution of base surge deposits. Based on co-eruptive modeling of Bato et al. ( 2021 ), the magma reservoir at \(\sim \) 5–6 km depth below TVI lost an estimated volume of −0.531 ± 0.004 km 3 . Much of this withdrawn magma is believed to have stalled at depth and emplaced as a northeast-striking dike extending under the towns of Taal, Lemery, Agoncillo, and San Nicolas, Batangas (Bato et al. 2021 ). Had most of the magma withdrawn from the reservoir been erupted in the 2020 event, it would still be classified as a VEI 4 with a more extensive pyroclastic surge deposit.
Maximum particle size data of the tephra deposits suggest that the 2020 eruption was larger than a “strong to moderate” event classified by PHIVOLCS at the time of eruption. We modeled an eruption height of 17.8 km using the Weibull method of TephraFits , which is consistent with the range of maximum heights measured from satellite images by Perttu et al. ( 2020 ) (16–17 km) and Bachmeier ( 2020 ) (20–21 km). Typical uncertainties of 20 % for determining plume heights (Bonadonna and Costa 2013 ) yield a maximum value of 21.26 km. This calculation matches the above-anvil cirrus plume temperature measurement of \(\sim 60^{\underline {\circ }}\) C, which translates to approximately 21 km based on comparison of data with 3 rawinsonde sites in Legaspi, Mactan, and Laoag, Philippines (Bachmeier 2020 ). The calculations of thickness half-distance b t and the ratio of thickness half-distance over thickness half-clast ( \(\frac {b_{t}}{b_{c}}\) ) plot in the sub-plinian classification scheme of Pyle ( 1989 ). A sub-plinian classification was also calculated using the classification scheme of Bonadonna and Costa ( 2013 ).
Grain size distribution, morphology, and composition
The volcanic glass component of the tephra deposits represents newly erupted magma that came into contact with the MCL. Recent studies suggest that explosive magma-water interactions can be identified in some tephra features, such as grain size, componentry, morphology, and texture (e.g., Wohletz ( 2013 ), Jordan et al. ( 2014 ), Thivet et al. ( 2020 ), and Ross et al. ( 2021 )). Nevertheless, phreatomagmatism fragmentation is still difficult to assess without any direct observation of the eruption itself (White and Valentine 2016 ). Present knowledge and monitoring of the Taal Volcano undoubtedly confirm the presence of a water lake (MCL) within the 2020 eruptive site. We also suggest that magma-water interaction favor (1) the fragmentation of some lithic grains (10–17% of the counted grains), (2) the occurrence of specific textural signatures (ubiquitous hackle lines, stepped features, and micro-fractures), and (3) the relatively fine grain size of the deposit (grain modes ranging between 90 and 500 μm depending on the deposit locations). Lithic grains, including hydrothermally altered fragments, sub-rounded volcanic rock fragments, and oxidized grains are those plucked from the volcano’s conduit during the eruption. Free crystals found in the tephra fall deposit were formed earlier in the magma body’s ascent history and liberated from the glassy matrix during eruption fragmentation. A phreatomagmatic eruption starting at about 4:00–5:30 p.m. of 12 January resulted in wet tephra to fall in clumps (Fig. 12 ). The excess water included in the eruption may have contributed to the dramatic display of thousands of lightning strokes and bolts (Prata et al. 2020 ; Van Eaton et al. 2020 ) (Fig. 12 b) throughout the period of the sub-plinian eruption.
Accretionary lapilli were abundant in the tephra fall deposits, especially in the areas of Agoncillo, Laurel, and San Nicolas Batangas (8–9 km away). In more distal areas, including Metro Manila (65 km away), tephra fall deposits include irregular-shaped aggregates with no specific internal grain organization. The “wet” nature of the eruption can be attributed to the interaction of the eruptive materials with the 42 million m 3 of water contained in the MCL (Bernard A et al. 2020 ) as evidenced by lapilli-sized aggregates. This is consistent with the interpretation of a phreatomagmatic eruption starting at about 4:00–5:30 p.m. of 12 January, which resulted in wet tephra to fall in clumps (Fig. 12 ). Base surges, known to form from the interaction of magma and water, are also reported to have cascaded down the slopes of Taal Volcano. They may have travelled across the lake up to about 600 m beyond the island’s shores based on the energy-line model (Lagmay et al. 2021 ).
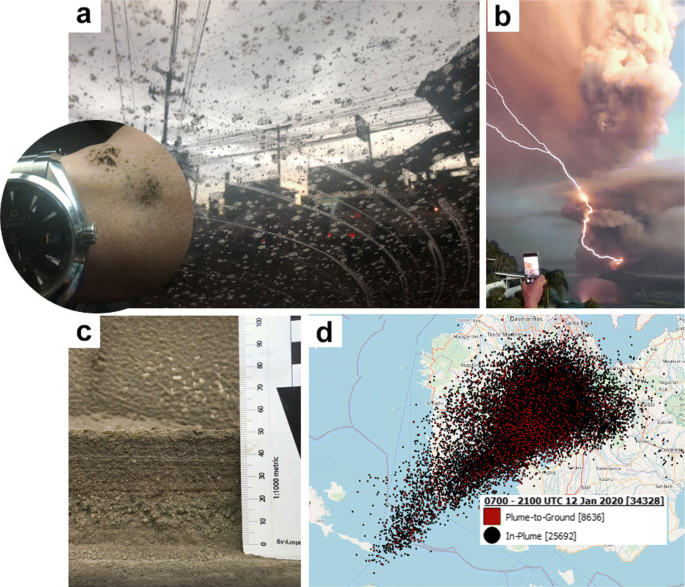
Evidence for a wet tephra plume. (a) Tephra falling in clumps on the car windshield in Silang, Cavite, 22 km north of Taal Volcano (inset shows clumps of tephra falling on the arm of author at 5:30 p.m. on 12 January). (b) Lightning during the eruption CC-BY Wikicommons. (c) Accretionary lapilli in tephra fall deposit. (d) Lightning produced during the eruption of Taal Volcano between 3:00 p.m. on 12 January and 5:00 a.m. on 13 January local time (0700–2100 UTC on 12 January). Red squares indicate plume-to-ground strokes; black circles indicate in-plume pulses. Courtesy of Chris Vagasky, Vaisala (Global Volcanism Program 2020 )
Composition of juvenile fragments
The glass fraction of juvenile tephra is andesitic in composition for the two samples that were analyzed. The whole-rock geochemistry of lava flows and loose rocks from the Volcano Island reported by Miklius et al. ( 1991 ) is plotted on the Le Maitre et al. ( 2005 ) TAS diagram showing most of the older eruption products were basalt to basaltic andesite in composition (Fig. 11 ). The 2020 microlite-free juvenile tephra materials are more andesitic and contain higher SiO 2 (55–56%) and Na 2 O + K 2 O (< 5.5%) than most of the older volcanic rocks. As a preliminary interpretation, this suggests that the magma erupted during the 2020 eruption is slightly more evolved relative to the 1968–1969 lavas erupted from Mt Tabaro, located southwest of Taal Volcano Island. However, for loose samples collected from the Main Crater rim and lavas from the Bignay eruption center, the composition is quite similar to the 2020 tephra (Fig. 11 ). A more detailed study of the 2020 eruptive products is the topic of another paper in preparation that will compare whole-rock geochemistry from different eruptive events.
Tephra fall (ashfall) hazards
The eruption of Taal Volcano deposited heavy, corrosive ash onto exposed surfaces such as house fittings (i.e., external pipes and gutters) and roads (Fig. 13 a). The interior of buildings were also affected by wind-blown ash, causing massive disruption of services (e.g., suspending work and school) and posing hazards to the health of residents (Fig. 13 b) (Baxter et al. 1982 ). In more severe cases, such as in the municipalities of Agoncillo and Laurel in Batangas, houses made of light materials collapsed as wet ash increased the load on roofs and walls (Fig. 13 c).
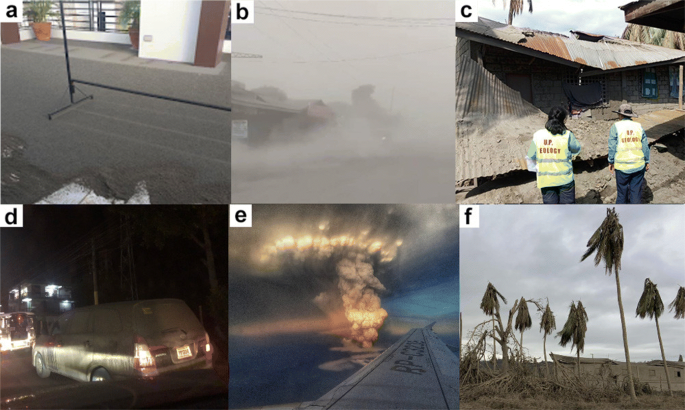
Tephra fall impacts. Ashfall (a) blanketing a garage in Laguna Province; (b) covering road surfaces; (c) causing the collapse of roofs; (d) impairing road visibility; (e) causing danger to inbound flights to Manila; and (f) damaging crops
Ground transportation was heavily impacted as primary and resuspended ash dispersed in air and caused reduced visibility and traction of road networks (Fig. 13 d). To wash off ashfall, cars needed to automatically spray water while wiping. Without spraying water, ash remained on the windshield and scratched the glass, reducing visibility for the car driver. The water tank for the wiper spray needed to be replenished after only a few minutes. For aviation, several airports including the Ninoy Aquino International Airport (NAIA) had to suspend over 240 flights (Rappler 2020 ; Reuters 2020 ; Chen 2020 ) as flying through ash clouds (Fig. 13 e) is known to cause significant damage to aircraft engines (Casadevall 1992 ; Guffanti et al. 2010 ).
Agriculture areas were also damaged by extensive tephra fall. Within the municipality of Agoncillo in Batangas, the leaves of coconut trees sagged and were on occasion felled by ashfall (Fig. 13 f). Pineapple crops and trees in Tagaytay, Cavite, were blanketed by ash for weeks. Taal Volcano’s eruption caused severe damage to the Philippines’ agricultural sector, with losses climbing to PhP 3.06 billion (USD 59.98 million/ EUR 54.19 million) according to the Department of Agriculture (CNN Philippines Staff 2020 ). Affected were coffee, cacao, pineapple, rice, coconut, and other high-value produce. The fisheries sector recorded the highest value of damage after the eruption, as it lost PhP 1.6 billion (USD 31.36 million/EUR 28.33 million) for the tilapia and milkfish (bangus) culture around the Taal Lake (CNN Philippines Staff 2020 ).
The National Disaster Risk Reduction and Management Council (NDRRMC) ordered the evacuation of at least 18,187 residents and housed them in 76 evacuation centers (MMDA 2020 ) because of the threats posed by the eruption and from ashfall. People were advised by the Department of Health (DOH) to stay indoors and wear medical masks to avoid inhalation of ash particles and skin exposure (Viray 2020 ).
Conclusions
On 12 January 2020, Taal Volcano erupted after 43 years of dormancy. Within a few hours from the start of the event at about 1 p.m., a towering 17–21-km-high eruption plume formed with its umbrella cloud drifting towards the north–northeast, affecting nearby provinces, including Metro Manila, the National Capital Region of the Philippines. Tephra fall deposits mantled the ground surface, covering an area of 8605 km 2 as far as 70 km north–northeast of Taal Volcano.
Tephra fall deposits were mapped using crowdsourced data collected during the 12–13 January main eruption, and field surveys within weeks after the peak eruptive period. This complementary technique for gathering data is particularly useful for a tropical country where tephra fall deposits are easily washed away by rain.
The TephraFits model results show that the tephra fall thins downwind exponentially with a thickness half-distance of about 1.40 km and 9.49 km for the proximal and distal exponential segments, respectively. In terms of tephra fall volume, model results using the exponential, power-law, and Weibull models, yield values of 0.057 km 3 , 0.042 km 3 , and 0.090 km 3 , respectively; all of these values translate to a VEI of 3.
However, using the probabilistic approach (Weibull method) with 90% confidence interval, the volume estimate can be as high as 0.097 km 3 . With the addition of the base surge deposits of 0.019 km 3 (Lagmay et al. 2021 ), the total eruption volume translates to a VEI of 4, which is more consistent with the classification for the observed plume height of 17–21 km (Perttu et al. 2020 ; Bachmeier 2020 ) and umbrella radius of Taal Volcano’s main eruption in 2020. A VEI of 4 is also consistent with the calculated median height of 17.8 km and sub-plinian classification which are based on the combined analysis of isopleth and isopach data derived from crowdsourced and field data.
The eruptive vent, located at the MCL, and the numerous fissures generated by ground deformation, allowed the explosive interaction of magma and water. Analyses of the fall deposits using binocular and electron microscopes reveal vitric (83–90%), lithic (7–11%), and crystal components (< 6%) that indicate a phreatomagmatic eruption. This interpretation is supported by the presence of accretionary lapilli in fall deposit layers and tephra falling in wet clumps at the height of the eruptive event. Textural signatures on juvenile particles, such as stepped features, also support the occurrence of the interaction between magma and external fluids at the fragmentation level, which enhanced the formation of fine ash particles.
Although the primary eruption lasted for only about 10 h, the damage to infrastructure and crops, and effects on air quality were significant. Eruptive activity continued for 7 days but was mainly characterized by discrete cannon-like explosions that generated 2-km-high bent-over plumes on 13 January that drifted to the west and southwest. Waning of activity generated much lower eruption heights of about 500 m until the eruptive activity ended on 22 January (PHIVOLCS 2020a ; 2020b ).
This study is a culmination not only of the authors’ own work, but also of the contributions of hundreds of members of the general public who stepped up to the call of citizen science, providing invaluable data and making themselves part of this crucial scientific work. Through their work and our own, we advance the knowledge on Taal Volcano, the second most active eruptive center in the Philippines with a long history of devastating eruptions. The growing density of population and rapid development of areas surrounding Taal Volcano make such understanding of its consequent tephra fall hazards and their impacts more significant.
Anthony R, Ringler A, Wilson D, Wolin E (2018) Do low-cost seismographs perform well enough for your network? an overview of laboratory tests and field observations of the OSOP Raspberry Shake 4D. Seismol Res Lett 90(1):219–228. https://doi.org/10.1785/0220180251
Article Google Scholar
Aurelio M, Lagmay A, Escudero J, Catugas S (2020a) Another large earthquake strikes the southern Philippines. Temblor, p 1. https://doi.org/10.32858/temblor.196
Aurelio M, Lagmay A, Escudero J, Catugas S (2020b) Latest Philippine earthquake reveals tectonic complexity. Temblor, p 1. https://doi.org/10.32858/temblor.191
Bachmeier S (2020) Eruption of the Taal Volcano in the Philippines. CIMSS Satellite Blog. https://bit.ly/3sm17Nn
Baclig C (2020) Out of 45 countries, PH ranks first as most obsessed with social media. Philippine Daily Inquirer. https://bit.ly/3kkb627
Bato MG, Lundgren P, Pinel V, Jr RS, Daag A, Cahulogan M (2021) The 2020 eruption and large lateral dike emplacement at Taal Volcano, Philippines: insights from satellite radar data. Geophysical Research Letters. https://doi.org/10.1029/2021GL092803
Baxter P, Bernstein R, Falk H, French J (1982) Medical aspects of a volcanic disaster: an outline of the hazards and emergency response measures. Disasters 6 (4):268–276. https://doi.org/10.1111/j.1467-7717.1982.tb00549.x
Bent A, Cassidy J, Prépetit C, Lamontagne M, Ulysse S (2018) Real-time seismic monitoring in Haiti and some applications. Seismol Res Lett 89(2A):407–415
Bernard A, Villacorte E, Maussen K, Caudron C, Robic J, Maximo R, Rebadulla R, Bornas MA, Solidum Jr R (2020) Carbon dioxide in taal volcanic lake: a simple gasometer for volcano monitoring, vol 47
Biass S, Bagheri G, Aeberhard WH, Bonadonna C (2014) Terror: towards a better quantification of the uncertainty propagated during the characterization of tephra deposits, statistics in volcanology. Statistics in Volcanology 1(2). https://doi.org/10.5038/2163-338X.1.2
Biass S, Bonadonna C, Houghton BF (2019) A step-by-step evaluation of empirical methods to quantify eruption source parameters from tephra-fall deposits. Journal of Applied Volcanology 8(1):1–16. https://doi.org/10.1186/s13617-018-0081-1
Bonadonna C, Costa A (2012) Estimating the volume of tephra deposits: a new simple strategy. Geol 40(5):415–418. https://doi.org/10.1130/G32769.1
Bonadonna C, Costa A (2013) Plume height, volume, and classification of explosive volcanic eruptions based on the Weibull function. Bull Volcanol 75(8):1–19. https://doi.org/10.1007/s00445-013-0742-1
Bonadonna C, Houghton B (2005) Total grain-size distribution and volume of tephra-fall deposits. Bull Volcanol 67(5):441–456. https://doi.org/10.1007/s00445-004-0386-2
Bonadonna C, Ernst G, Sparks R (1998) Thickness variations and volume estimates of tephra fall deposits: the importance of particle Reynolds number. J Volcanol Geotherm Res 81(3):173–187. https://doi.org/10.1016/S0377-0273(98)00007-9
Casadevall T (1992) Volcanic hazards and aviation safety: lessons of the past decade. Federal Aviation Administration Aviation Safety Journal 2:3–11
Google Scholar
Chen J (2020) Manila Airport partially reopens after Taal volcano eruption leads to over 240 cancelled flights. Business Traveller Asia Pacific https://bit.ly/3ma2f5q
CNN Philippines Staff (2020) Agricultural damage from Taal eruption hits Php 3.06-B. CNN Philippines https://bit.ly/3smrLWr
Congress of the Philippines (2020) Republic Act 9500, The University of the Philippines Charter of 2008. https://www.officialgazette.gov.ph/2008/04/19/republic-act-no-9500/
Constantinescu R, Hopulele-Gligor A, Connor CB, Bonadonna C, Connor LJ, Lindsay JM, Charbonnier S, Volentik AC (2021) The radius of the umbrella cloud helps characterize large explosive volcanic eruptions. Communications Earth & Environment 2(1):1–8. https://doi.org/10.1038/s43247-020-00078-3
Daggitt ML, Mather T, Pyle DM, Page S (2014) AshCalc–a new tool for the comparison of the exponential, power-law and Weibull models of tephra deposition. Journal of Applied Volcanology 3(7). https://doi.org/10.1186/2191--5040--3--7
Del Castillo M, Paraiso P, Vicente M, Jamero M, Narisma G (2020) Impacts of Taal Volcano phreatic eruption (12 January 2020) on the environment and population: satellite-based observations compared with historical records. https://bit.ly/3kdAxCh
Fierstein J, Nathenson M (1992) Another look at the calculation of fallout tephra volumes. Bull Volcanol 54(2):156–167. https://doi.org/10.1007/BF00278005
Global Volcanism Program (2020) Report on Taal (Philippines) Krippner JB, Venzke E (eds). Bulletin of the Global Volcanism Network, vol 45 No. 6. Smithsonian Institution. https://doi.org/10.5479/si.GVP.BGVN202006-273070
Guffanti M, Casadevall T, Budding K (2010) Encounters of aircraft with volcanic ash clouds; A compilation of known incidents, 1953–2009. US Geological Survey Data 545:12. https://pubs.usgs.gov/ds/545/
Gurioli L, Di Muro A, Vlastélic I, Moune S, Thivet S, Valer M, Villeneuve N, Boudoire G, Peltier A, Bachèlery P et al (2018) Integrating field, textural, and geochemical monitoring to track eruption triggers and dynamics: a case study from Piton de la Fournaise. Solid Earth 9(2):431. https://doi.org/10.5194/se-9-431-2018
Holmgren J, Werner M (2021) Raspberry shake instruments provide initial ground-motion assessment of the induced seismicity at the United Downs Deep Geothermal Power Project in Cornwall, United Kingdom. The Seismic Record 1(1):27–34. https://doi.org/10.1785/0320210010
International ASTM (2014) Standard Specification for Woven Wire Test Sieve Cloth and Test Sieves. ASTM International, West Conshocken, PA
Jordan S, Dürig T, Cas R, Zimanowski B (2014) Processes controlling the shape of ash particles: Results of statistical IPA. J Volcanol Geotherm Res 288:19–27. https://doi.org/10.1016/j.jvolgeores.2014.09.012
Lagmay A, Balangue-Tarriela R, Aurelio M, Ybanez R, Ybanez A, Sulapas J, Baldago M, Sarmiento D, Cabria H, Rodolfo R, Rafael D, Trinidad R, Obille E, Rosell N (2021) Hazardous base surges of taal’s 2020 eruption. Scientific Reports, 11(15703). https://doi.org/10.1038/s41598-021-94866-2
Le Maitre RW, Streckeisen A, Zanettin B, Le Bas M, Bonin B, Bateman P (2005) Igneous rocks: a classification and glossary of terms: recommendations of the International Union of Geological Sciences Subcommission on the Systematics of Igneous Rocks. Cambridge University Press, Cambridge
Legros F (2000) Minimum volume of a tephra fallout deposit estimated from a single isopach. J Volcanol Geotherm Res 96(1-2):25–32. https://doi.org/10.1016/S0377-0273(99)00135-3
Miklius A, Flower M, Huijmans J, Mukasa S, Samuel B, Castillo P (1991) Geochemistry of lavas from Taal Volcano, southwestern Luzon, Philippines: evidence for multiple magma supply systems and mantle source heterogeneity. J Petrol 32(3):593–627. https://doi.org/10.1093/petrology/32.3.593
MMDA (2020) MMDA to Set Up Portable Water Purifiers in Batangas. https://bit.ly/3xTccGE
Perttu A, Taisne B, De Angelis S, Assink JD, Tailpied D, Williams RA (2020) Estimates of plume height from infrasound for regional volcano monitoring. J Volcanol Geotherm Res 106997:402. https://doi.org/10.1016/j.jvolgeores.2020.106997
PHIVOLCS (2020a) Taal Volcano Bulletin 12 January 2020 04:00 PM. https://bit.ly/3z7Fxil
PHIVOLCS (2020b) Taal Volcano Bulletin 26 January 2020. https://bit.ly/3AQY1nk
Prata A, Folch A, Prata A, Biondi R, Brenot H, Cimarelli C, Corradini S, Lapierre J, Costa A (2020) Anak krakatau triggers volcanic freezer in the upper troposphere. Scientific Reports 10(1):1–13
PSA (2021) 2020 Census of Population and Housing (2020 CPH) Population Counts Declared Official by the President. https://bit.ly/3xXPjBV
PTCC (2020) Are you affected? https://fb.watch/4mw1qqclqI/
Pyle DM (1989) The thickness, volume and grainsize of tephra fall deposits. Bull Volcanol 51 (1):1–15. https://doi.org/10.1007/BF01086757
Pyle DM (1990) New estimates for the volume of the Minoan eruption. Thera and the Aegean World III(2):113–121
Pyle DM (1995) Assessment of the minimum volume of tephra fall deposits. J Volcanol Geotherm Res 69(3-4):379–382. https://doi.org/10.1016/0377-0273(95)00038-0
Pyle DM (1999) Widely dispersed quaternary tephra in Africa. Glob Planet Chang 21(1-3):95–112. https://doi.org/10.1016/S0921-8181(99)00009-0
Rappler (2020) NAIA flights ’on hold’ due to Taal volcano ash eruption. Rappler. https://bit.ly/3xWvY46
Reuters (2020) Philippines suspends Manila airport flights as volcano spews ash. Reuters. https://reut.rs/3yVxiFV
Ross P, Dürig T, Comida P, Lefebvre N, White J, Andronico D, Thivet S, Eychenne J, Gurioli L (2021) Standardized analysis of juvenile pyroclasts in comparative studies of primary magma fragmentation; 1. Overview and workflow. Bull Volcanol 84(13). https://doi.org/10.1007/s00445-021-01516-6
Subedi S, Hetényi G, Denton P, Sauron A (2020) Seismology at school in Nepal: a program for educational and citizen seismology through a low-cost seismic network. Frontiers in Earth Science 8:73. https://doi.org/10.3389/feart.2020.00073
Sulpizio R (2005) Three empirical methods for the calculation of distal volume of tephra-fall deposits. J Volcanol Geotherm Res 145(3):315–336. https://doi.org/10.1016/j.jvolgeores.2005.03.001
Thivet S, Gurioli L, Di Muro A, Eychenne J, Besson P, Nedelec JM (2020) Variability of ash deposits at Piton de la Fournaise (La Reunion Island): insights into fragmentation processes at basaltic shield volcanoes. Bull Volcanol 82(9):1–20. https://doi.org/10.1007/s00445-020-01398-0
Torres R, Self S, Punongbayan R (1995) Attention focuses on taal: Decade volcano of the philippines. Eos, Transactions American Geophysical Union 76(24):241–247
Van Eaton AR, Schneider DJ, Smith CM, Haney MM, Lyons JJ, Said R, Fee D, Holzworth RH, Mastin LG (2020) Did ice-charging generate volcanic lightning during the 2016–2017 eruption of bogoslof volcano, alaska? Bull Volcanol 82(3):1–23
Viray PL (2020) DOH cautions public on health effects of Taal ashfall. Philstar Global. https://bit.ly/2XAp0W7
Watt SF, Pyle DM, Mather TA, Martin RS, Matthews NE (2009) Fallout and distribution of volcanic ash over Argentina following the May 2008 explosive eruption of chaitén, Chile. Journal of Geophysical Research: Solid Earth, 114(B4). https://doi.org/10.1029/2008JB006219
White J, Valentine G (2016) Magmatic versus phreatomagmatic fragmentation: absence of evidence is not evidence of absence. Geosphere 12:1478–1488. https://doi.org/10.1130/GES01337.1
Wohletz K (2013) Magma–water interactions. Cambridge University Press, Cambridge. https://doi.org/10.1017/CBO9781139021562.011
Book Google Scholar
Download references
Acknowledgements
We thank the citizen scientists who contributed their records, donated samples of the ashfall deposits in their neighborhood, helped in the processing of samples for analysis, and participated in the editing of the draft manuscript. The authors greatly appreciate the efforts of Dr. Peter Julian Cayton, who shared his statistical expertise, and Mr. Eligio Obille Jr., who took time to review the manuscript. We also thank Steve Self, Kristi Wallace, and Michael Ort for their insightful reviews.
This work is funded by the National Institute of Geological Sciences and the UP Resilience Institute project on complex hazards. It is part of the Geoheritage for Geohazard Resilience (UNESCO Project 692) which aims to empower areas with the capacity to communicate and manage geohazards and to establish a global community for best practice in Geoheritage for Geohazard Resilience.
Author information
Authors and affiliations.
UP National Institute of Geological Sciences, College of Science, University of the Philippines, Diliman, Quezon City, Metro Manila, Philippines
M. I. R. Balangue-Tarriela, A. M. F. Lagmay, D. M. Sarmiento, M. C. Baldago, R. Ybañez, J. R. Trinidad, M. Aurelio, D. J. Rafael & J. A. Escudero
UP Resilience Institute and NOAH Center, University of the Philippines, Diliman, Quezon City, Metro Manila, Philippines
A. M. F. Lagmay, J. Vasquez, A. A. Ybañez & A. Bermas
Laboratoire Magmas et Volcans, Université Clermont Auvergne, CNRS, IRD, OPGC, F-63000, Clermont-Ferrand, France
S. Thivet, L. Gurioli & B. Van Wyk de Vries
You can also search for this author in PubMed Google Scholar
Corresponding author
Correspondence to A. M. F. Lagmay .
Additional information
Editorial responsibility: M.H. Ort; Deputy Executive Editor: J. Tadeucci
Publisher’s Note
Springer Nature remains neutral with regard to jurisdictional claims in published maps and institutional affiliations.

Electronic supplementary material
Below is the link to the electronic supplementary material.
(MOV 21.8 MB)
Rights and permissions.
Open Access This article is licensed under a Creative Commons Attribution 4.0 International License, which permits use, sharing, adaptation, distribution and reproduction in any medium or format, as long as you give appropriate credit to the original author(s) and the source, provide a link to the Creative Commons licence, and indicate if changes were made. The images or other third party material in this article are included in the article's Creative Commons licence, unless indicated otherwise in a credit line to the material. If material is not included in the article's Creative Commons licence and your intended use is not permitted by statutory regulation or exceeds the permitted use, you will need to obtain permission directly from the copyright holder. To view a copy of this licence, visit http://creativecommons.org/licenses/by/4.0/ .
Reprints and permissions
About this article
Balangue-Tarriela, M.I.R., Lagmay, A.M.F., Sarmiento, D.M. et al. Analysis of the 2020 Taal Volcano tephra fall deposits from crowdsourced information and field data. Bull Volcanol 84 , 35 (2022). https://doi.org/10.1007/s00445-022-01534-y
Download citation
Received : 13 September 2021
Accepted : 14 January 2022
Published : 02 March 2022
DOI : https://doi.org/10.1007/s00445-022-01534-y
Share this article
Anyone you share the following link with will be able to read this content:
Sorry, a shareable link is not currently available for this article.
Provided by the Springer Nature SharedIt content-sharing initiative
- Crowdsourced
- Tephra fall
- Find a journal
- Publish with us
- Track your research
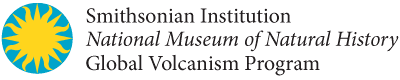
- Current Eruptions
- Smithsonian / USGS Weekly Volcanic Activity Report
- Bulletin of the Global Volcanism Network
- Weekly Report 20th Anniversary
- Holocene Volcano List
- Pleistocene Volcano List
- Country Volcano Lists
- Volcano Search
- Eruption Search
- Deformation Search
- Emission Search
- Webservices
- Database Information
- Image Collections
- Video Collections
- Theme Collections
- Keyword Collections
- St. Helens 40th Anniversary
- Frequent Questions
- Information Sources
- Google Earth Placemarks
- This Dynamic Planet
- Eruptions, Earthquakes & Emissions Application
- Volcano Numbers
- Volcano Naming
- How to Cite
- Terms of Use
Report on Taal (Philippines) — June 2020
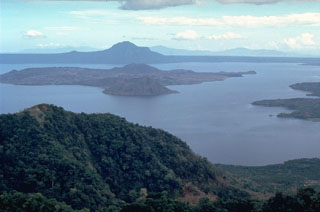
Bulletin of the Global Volcanism Network, vol. 45, no. 6 (June 2020) Managing Editor: Edward Venzke. Edited by Janine B. Krippner. Taal (Philippines) Eruption on 12 January with explosions through 22 January; steam plumes continuing into March
Please cite this report as: Global Volcanism Program, 2020. Report on Taal (Philippines) (Krippner, J.B., and Venzke, E., eds.). Bulletin of the Global Volcanism Network , 45:6. Smithsonian Institution. https://doi.org/10.5479/si.GVP.BGVN202006-273070
Philippines
14.0106°n, 120.9975°e; summit elev. 311 m, all times are local (unless otherwise noted).
Taal volcano is in a caldera system located in southern Luzon island and is one of the most active volcanoes in the Philippines. It has produced around 35 recorded eruptions since 3,580 BCE, ranging from VEI 1 to 6, with the majority of eruptions being a VEI 2. The caldera contains a lake with an island that also contains a lake within the Main Crater (figure 12). Prior to 2020 the most recent eruption was in 1977, on the south flank near Mt. Tambaro. The United Nations Office for the Coordination of Humanitarian Affairs in the Philippines reports that over 450,000 people live within 40 km of the caldera (figure 13). This report covers activity during January through February 2020 including the 12 to 22 January eruption, and is based on reports by Philippine Institute of Volcanology and Seismology (PHIVOLCS), satellite data, geophysical data, and media reports.
The hazard status at Taal was raised to Alert Level 1 (abnormal, on a scale of 0-5) on 28 March 2019. From that date through to 1 December there were 4,857 earthquakes registered, with some felt nearby. Inflation was detected during 21-29 November and an increase in CO 2 emission within the Main Crater was observed. Seismicity increased beginning at 1100 on 12 January. At 1300 there were phreatic (steam) explosions from several points inside Main Crater and the Alert Level was raised to 2 (increasing unrest). Booming sounds were heard in Talisay, Batangas, at 1400; by 1402 the plume had reached 1 km above the crater, after which the Alert Level was raised to 3 (magmatic unrest).
Phreatic eruption on 12 January 2020. A seismic swarm began at 1100 on 12 January 2020 followed by a phreatic eruption at 1300. The initial activity consisted of steaming from at least five vents in Main Crater and phreatic explosions that generated 100-m-high plumes. PHIVOLCS raised the Alert Level to 2. The Earth Observatory of Singapore reported that the International Data Center (IDC) for the Comprehensive test Ban Treaty (CTBT) in Vienna noted initial infrasound detections at 1450 that day.
Booming sounds were heard at 1400 in Talisay, Batangas (4 km NNE from the Main Crater), and at 1404 volcanic tremor and earthquakes felt locally were accompanied by an eruption plume that rose 1 km; ash fell to the SSW. The Alert Level was raised to 3 and the evacuation of high-risk barangays was recommended. Activity again intensified around 1730, prompting PHIVOLCS to raise the Alert Level to 4 and recommend a total evacuation of the island and high-risk areas within a 14-km radius. The eruption plume of steam, gas, and tephra significantly intensified, rising to 10-15 km altitude and producing frequent lightning (figures 14 and 15). Wet ash fell as far away as Quezon City (75 km N). According to news articles schools and government offices were ordered to close and the Ninoy Aquino International Airport (56 km N) in Manila suspended flights. About 6,000 people had been evacuated. Residents described heavy ashfall, low visibility, and fallen trees.
In a statement issued at 0320 on 13 January, PHIVOLCS noted that ashfall had been reported across a broad area to the north in Tanauan (18 km NE), Batangas; Escala (11 km NW), Tagaytay; Sta. Rosa (32 km NNW), Laguna; Dasmariñas (32 km N), Bacoor (44 km N), and Silang (22 km N), Cavite; Malolos (93 km N), San Jose Del Monte (87 km N), and Meycauayan (80 km N), Bulacan; Antipolo (68 km NNE), Rizal; Muntinlupa (43 km N), Las Piñas (47 km N), Marikina (70 km NNE), Parañaque (51 km N), Pasig (62 km NNE), Quezon City, Mandaluyong (62 km N), San Juan (64 km N), Manila; Makati City (59 km N) and Taguig City (55 km N). Lapilli (2-64 mm in diameter) fell in Tanauan and Talisay; Tagaytay City (12 km N); Nuvali (25 km NNE) and Sta (figure 16). Rosa, Laguna. Felt earthquakes (Intensities II-V) continued to be recorded in local areas.
Magmatic eruption on 13 January 2020. A magmatic eruption began during 0249-0428 on 13 January, characterized by weak lava fountaining accompanied by thunder and flashes of lightning. Activity briefly waned then resumed with sporadic weak fountaining and explosions that generated 2-km-high, dark gray, steam-laden ash plumes (figure 17). New lateral vents opened on the N flank, producing 500-m-tall lava fountains. Heavy ashfall impacted areas to the SW, including in Cuenca (15 km SSW), Lemery (16 km SW), Talisay, and Taal (15 km SSW), Batangas (figure 18).
News articles noted that more than 300 domestic and 230 international flights were cancelled as the Manila Ninoy Aquino International Airport was closed during 12-13 January. Some roads from Talisay to Lemery and Agoncillo were impassible and electricity and water services were intermittent. Ashfall in several provinces caused power outages. Authorities continued to evacuate high-risk areas, and by 13 January more than 24,500 people had moved to 75 shelters out of a total number of 460,000 people within 14 km.
A PHIVOLCS report for 0800 on the 13th through 0800 on 14 January noted that lava fountaining had continued, with steam-rich ash plumes reaching around 2 km above the volcano and dispersing ash SE and W of Main Crater. Volcanic lighting continued at the base of the plumes. Fissures on the N flank produced 500-m-tall lava fountains. Heavy ashfall continued in the Lemery, Talisay, Taal, and Cuenca, Batangas Municipalities. By 1300 on the 13th lava fountaining generated 800-m-tall, dark gray, steam-laden ash plumes that drifted SW. Sulfur dioxide emissions averaged 5,299 metric tons/day (t/d) on 13 January and dispersed NNE (figure 19).
Explosions and ash emission through 22 January 2020. At 0800 on 15 January PHIVOLCS stated that activity was generally weaker; dark gray, steam-laden ash plumes rose about 1 km and drifted SW. Satellite images showed that the Main Crater lake was gone and new craters had formed inside Main Crater and on the N side of Volcano Island.
PHIVOLCS reported that activity during 15-16 January was characterized by dark gray, steam-laden plumes that rose as high as 1 km above the vents in Main Crater and drifted S and SW. Sulfur dioxide emissions were 4,186 t/d on 15 January. Eruptive events at 0617 and 0621 on 16 January generated short-lived, dark gray ash plumes that rose 500 and 800 m, respectively, and drifted SW. Weak steam plumes rose 800 m and drifted SW during 1100-1700, and nine weak explosions were recorded by the seismic network.
Steady steam emissions were visible during 17-21 January. Infrequent weak explosions generated ash plumes that rose as high as 1 km and drifted SW. Sulfur dioxide emissions fluctuated and were as high as 4,353 t/d on 20 January and as low as 344 t/d on 21 January. PHIVOLCS reported that white steam-laden plumes rose as high as 800 m above main vent during 22-28 January and drifted SW and NE; ash emissions ceased around 0500 on 22 January. Remobilized ash drifted SW on 22 January due to strong low winds, affecting the towns of Lemery (16 km SW) and Agoncillo, and rose as high as 5.8 km altitude as reported by pilots. Sulfur dioxide emissions were low at 140 t/d.
Steam plumes through mid-April 2020. The Alert Level was lowered to 3 on 26 January and PHIVOLCS recommended no entry onto Volcano Island and Taal Lake, nor into towns on the western side of the island within a 7-km radius. PHIVOLCS reported that whitish steam plumes rose as high as 800 m during 29 January-4 February and drifted SW (figure 20). The observed steam plumes rose as high as 300 m during 5-11 February and drifted SW.
Sulfur dioxide emissions averaged around 250 t/d during 22-26 January; emissions were 87 t/d on 27 January and below detectable limits the next day. During 29 January-4 February sulfur dioxide emissions ranged to a high of 231 t/d (on 3 February). The following week sulfur dioxide emissions ranged from values below detectable limits to a high of 116 t/d (on 8 February).
On 14 February PHIVOLCS lowered the Alert Level to 2, noting a decline in the number of volcanic earthquakes, stabilizing ground deformation of the caldera and Volcano Island, and diffuse steam-and-gas emission that continued to rise no higher than 300 m above the main vent during the past three weeks. During 14-18 February sulfur dioxide emissions ranged from values below detectable limits to a high of 58 tonnes per day (on 16 February). Sulfur dioxide emissions were below detectable limits during 19-20 February. During 26 February-2 March steam plumes rose 50-300 m above the vent and drifted SW and NE. PHIVOLCS reported that during 4-10 March weak steam plumes rose 50-100 m and drifted SW and NE; moderate steam plumes rose 300-500 m and drifted SW during 8-9 March. During 11-17 March weak steam plumes again rose only 50-100 m and drifted SW and NE.
PHIVOLCS lowered the Alert Level to 1 on 19 March and recommended no entry onto Volcano Island, the area defined as the Permanent Danger Zone. During 8-9 April steam plumes rose 100-300 m and drifted SW. As of 1-2 May 2020 only weak steaming and fumarolic activity from fissure vents along the Daang Kastila trail was observed.
Evacuations. According to the Disaster Response Operations Monitoring and Information Center (DROMIC) there were a total of 53,832 people dispersed to 244 evacuation centers by 1800 on 15 January. By 21 January there were 148,987 people in 493 evacuation. The number of residents in evacuation centers dropped over the next week to 125,178 people in 497 locations on 28 January. However, many residents remained displaced as of 3 February, with DROMIC reporting 23,915 people in 152 evacuation centers, but an additional 224,188 people staying at other locations.
By 10 February there were 17,088 people in 110 evacuation centers, and an additional 211,729 staying at other locations. According to the DROMIC there were a total of 5,321 people in 21 evacuation centers, and an additional 195,987 people were staying at other locations as of 19 February.
The number of displaced residents continued to drop, and by 3 March there were 4,314 people in 12 evacuation centers, and an additional 132,931 people at other locations. As of 11 March there were still 4,131 people in 11 evacuation centers, but only 17,563 staying at other locations.
Deformation and ground cracks. New ground cracks were observed on 13 January in Sinisian (18 km SW), Mahabang Dahilig (14 km SW), Dayapan (15 km SW), Palanas (17 km SW), Sangalang (17 km SW), and Poblacion (19 km SW) Lemery; Pansipit (11 km SW), Agoncillo; Poblacion 1, Poblacion 2, Poblacion 3, Poblacion 5 (all around 17 km SW), Talisay, and Poblacion (11 km SW), San Nicolas (figure 21). A fissure opened across the road connecting Agoncillo to Laurel, Batangas. New ground cracking was reported the next day in Sambal Ibaba (17 km SW), and portions of the Pansipit River (SW) had dried up.
Dropping water levels of Taal Lake were first observed in some areas on 16 January but reported to be lake-wide the next day. The known ground cracks in the barangays of Lemery, Agoncillo, Talisay, and San Nicolas in Batangas Province widened a few centimeters by 17 January, and a new steaming fissure was identified on the N flank of the island.
GPS data had recorded a sudden widening of the caldera by ~1 m, uplift of the NW sector by ~20 cm, and subsidence of the SW part of Volcano Island by ~1 m just after the main eruption phase. The rate of deformation was smaller during 15-22 January, and generally corroborated by field observations; Taal Lake had receded about 30 cm by 25 January but about 2.5 m of the change (due to uplift) was observed around the SW portion of the lake, near the Pansipit River Valley where ground cracking had been reported.
Weak steaming (plumes 10-20 m high) from ground cracks was visible during 5-11 February along the Daang Kastila trail which connects the N part of Volcano Island to the N part of the main crater. PHIVOLCS reported that during 19-24 February steam plumes rose 50-100 m above the vent and drifted SW. Weak steaming (plumes up to 20 m high) from ground cracks was visible during 8-14 April along the Daang Kastila trail which connects the N part of Volcano Island to the N part of the main crater.
Seismicity. Between 1300 on 12 January and 0800 on 21 January the Philippine Seismic Network (PSN) had recorded a total of 718 volcanic earthquakes; 176 of those had magnitudes ranging from 1.2-4.1 and were felt with Intensities of I-V. During 20-21 January there were five volcanic earthquakes with magnitudes of 1.6-2.5; the Taal Volcano network (which can detect smaller events not detectable by the PSN) recorded 448 volcanic earthquakes, including 17 low-frequency events. PHIVOLCS stated that by 21 January hybrid earthquakes had ceased and both the number and magnitude of low-frequency events had diminished.
Geological Summary. Taal is one of the most active volcanoes in the Philippines and has produced some powerful eruptions. The 15 x 20 km Talisay (Taal) caldera is largely filled by Lake Taal, whose 267 km2 surface lies only 3 m above sea level. The maximum depth of the lake is 160 m, with several submerged eruptive centers. The 5-km-wide Volcano Island in north-central Lake Taal is the location of all observed eruptions. The island is composed of coalescing small stratovolcanoes, tuff rings, and scoria cones. Powerful pyroclastic flows and surges have caused many fatalities.
Information Contacts: Philippine Institute of Volcanology and Seismology (PHIVOLCS) , Department of Science and Technology, University of the Philippines Campus, Diliman, Quezon City, Philippines (URL: http://www.phivolcs.dost.gov.ph/); Disaster Response Operations Monitoring and Information Center (DROMIC) (URL: https://dromic.dswd.gov.ph/); United Nations Office for the Coordination of Humanitarian Affairs , Philippines (URL: https://www.unocha.org/philippines); James Reynolds , Earth Uncut TV (Twitter: @EarthUncutTV, URL: https://www.earthuncut.tv/, YouTube: https://www.youtube.com/user/TyphoonHunter); Chris Vagasky , Vaisala Inc., Louisville, Colorado, USA (URL: https://www.vaisala.com/en?type=1, Twitter: @COweatherman, URL: https://twitter.com/COweatherman); Earth Observatory of Singapore , Nanyang Technological University, 50 Nanyang Avenue, Singapore (URL: https://www.earthobservatory.sg/); Global Sulfur Dioxide Monitoring Page , Atmospheric Chemistry and Dynamics Laboratory, NASA Goddard Space Flight Center (NASA/GSFC), 8800 Greenbelt Road, Goddard, Maryland, USA (URL: https://so2.gsfc.nasa.gov/); Relief Web , Flash Update No. 1 - Philippines: Taal Volcano eruption (As of 13 January 2020, 2 p.m. local time) (URL: https://reliefweb.int/report/philippines/flash-update-no-1-philippines-taal-volcano-eruption-13-january-2020-2-pm-local); Bloomberg , Philippines Braces for Hazardous Volcano Eruption (URL: https://www.bloomberg.com/news/articles/2020-01-12/philippines-raises-alert-level-in-taal-as-volcano-spews-ash); National Public Radio (NPR) , Volcanic Eruption In Philippines Causes Thousands To Flee (URL: npr.org/2020/01/13/795815351/volcanic-eruption-in-philippines-causes-thousands-to-flee); Reuters (http://www.reuters.com/); Agence France-Presse (URL: http://www.afp.com/); Pacific Press (URL: http://www.pacificpress.com/); Shutterstock (URL: https://www.shutterstock.com/); Getty Images (URL: http://www.gettyimages.com/); Google Earth (URL: https://www.google.com/earth/).

NASA Applied Sciences
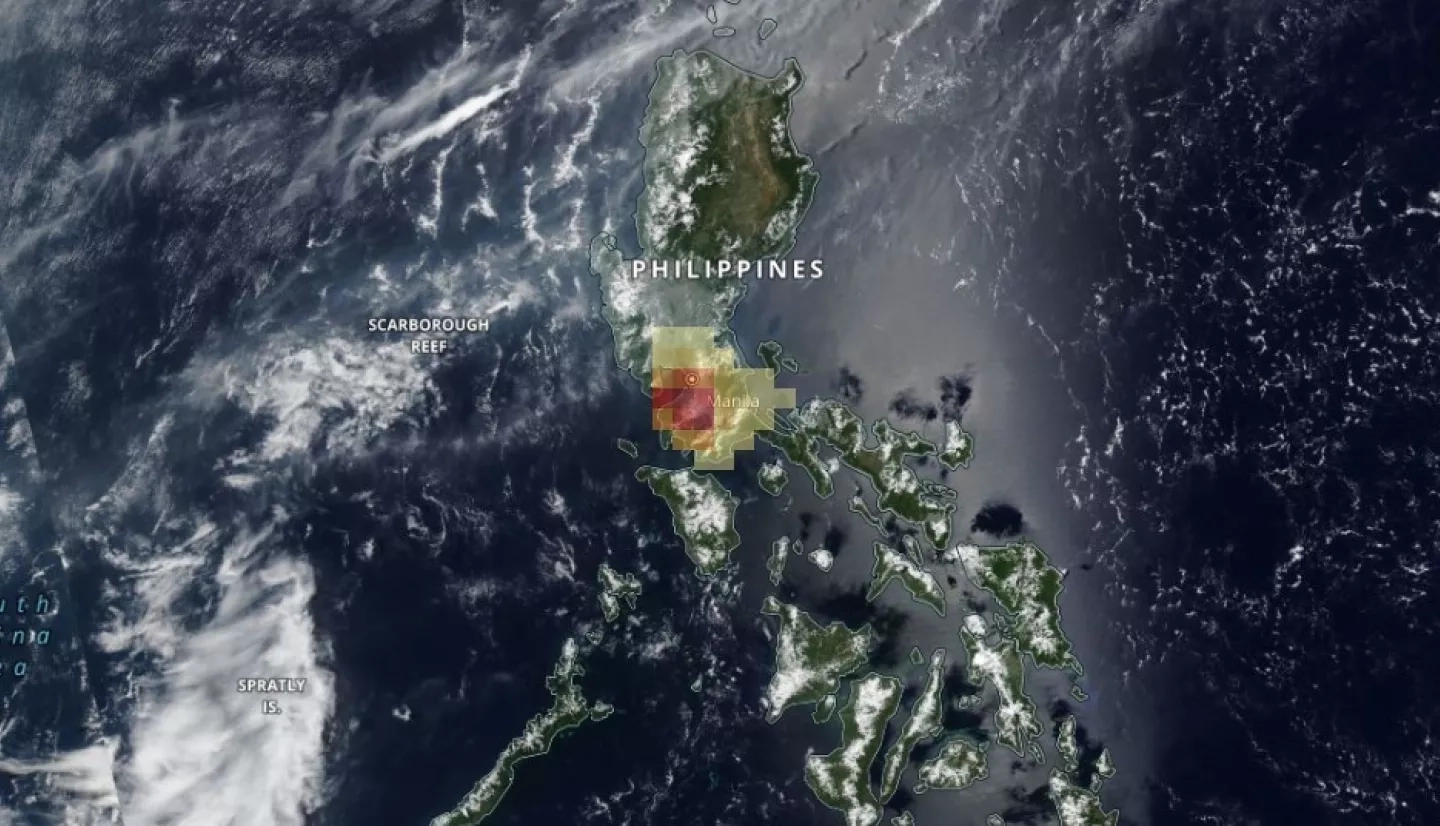
Taal Volcanic Eruption 2021
In late June 2021, Taal Volcano, located in the province of Batangas in the Philippines, has experienced increased volcanic activity, prompting the evacuation of thousands from surrounding towns. Taal had been dormant for 43 years until it became active again in January 2020 .
In early July 2021, Taal experienced a series of phreatomagmatic eruptions – explosive interactions of water and magma. On July 7 the Taal Volcano Network reported 55 volcanic earthquakes in a span of 24 hours, and the volcano released high levels of sulfur dioxide into the air. All travel to the Taal Volcano Island is prohibited due to the risk of pyroclastic density currents and volcanic tsunamis, and nearby communities are advised to remain vigilant and prepare for evacuation if necessary. Currently, the primary concerns are impacts to local air quality and the potential for another stronger eruption to occur. Although Taal is one of the world’s smallest active volcanoes, it still has the potential to be deadly – a 1911 eruption of Taal killed over 1300 people.
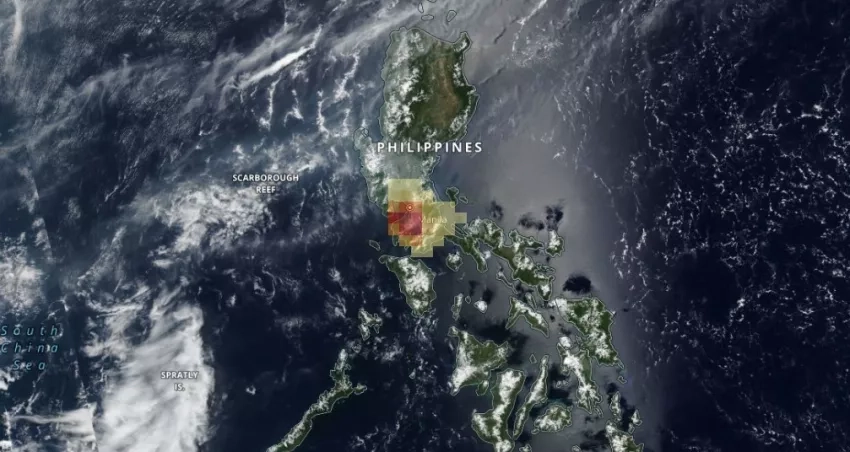
The NASA Earth Applied Sciences Disasters program area has activated efforts to monitor Taal Volcano with Earth-observing data for air quality concerns, surface deformation, or any signs that could indicate the potential for a larger eruption. The Disasters program is working with the USGS Volcano Disaster Assistance Program ( VDAP ), which is supporting regional stakeholders including the Philippine Institute of Volcanology and Seismology ( PHIVOLCS ) and USAID’s Bureau of Humanitarian Assistance ( BHA ). Researchers from the Earth Surface and Interior Group at NASA’s Jet Propulsion Laboratory and the University of Pittsburgh are working with the Disasters program to provide data and analysis. Together they are using OMPS data to monitor sulfur dioxide emissions, ASTER data to monitor volcanic hot spots, and SAR data to monitor surface deformation.
The NASA ROSES A.37 research project “ Integrating SAR Data for Improved Resilience and Response to Weather-Related Disasters ” is providing support for the eruption, and the “ Day-Night Monitoring of Volcanic SO2 and Ash for Aviation Avoidance at Northern Polar Latitudes: Enhancing Direct Readout capabilities from EOS, SNPP and NOAA20 ” A.37 research project produced multiple maps that depict sulfur dioxide levels over the Philippines from before, during, and after the eruption.
By combining analysis from multiple satellites and sensors and coordinating with experts throughout the disaster research community, the NASA Disasters program area can monitor the volcanic activity as an integrated system, and equip local stakeholders with the tools they need to better prepare for a potential eruption and mitigate its impacts.
Learn more about how NASA supports risk reduction, response and recovery for volcanic eruptions.
Previous Volcano Activations:
- Mt. Nyiragongo Eruption 2021
- Saint Vincent and Martinique Volcanic Activity 2021
- Guatemala Volcanic Activity 2021
Connect with the Disasters Program
With help from NASA’s Earth-observing satellites, our community is making a difference on our home planet. Find out how by staying up-to-date on their latest projects and discoveries.
Stay Connected
taal volcano Recently Published Documents
Total documents.
- Latest Documents
- Most Cited Documents
- Contributed Authors
- Related Sources
- Related Keywords
Supplemental Material: Eruption dynamics leading to a volcanic thunderstorm—The January 2020 eruption of Taal volcano, Philippines
Expanded methodology and calculations, plume modeling, and details of lightning data and photographs.<br>
INSAR-BASED LOS SURFACE DEFORMATION COMPARISON OF METRO MANILA BEFORE AND AFTER THE JANUARY 2020 TAAL VOLCANO ERUPTION
Abstract. The city of Metro Manila has been constantly battered by several hazards on an annual basis. On January 2020, the Taal Volcano erupted with multiple recorded earthquakes. Previous literatures have found that Metro Manila is experiencing a steady subsidence. Determination of land uplift or subsidence is crucial in planning and mitigating the effects of flooding in the area. The study aims to determine whether an uplift occurred in Metro Manila after the eruption or is the study area still experiencing subsidence This study uses a pair L1 SLC Sentinel 1 Images. Radar Interferometry is used to generate Interferograms and Satellite Line of Sight (LOS) deformation was determined between the 2 dates of image acquisition. It was found that the Metro Manila area generally experienced an uplift except for some areas in Caloocan which shows subsidence. The uplift magnitude gradually decreases going from the South to North with a max value of 9.6 cm.
ASHFALL DISPERSAL MAPPING OF THE 2020 TAAL VOLCANO ERUPTION USING DIWATA-2 IMAGERY FOR DISASTER ASSESSMENT
Abstract. Natural disasters incur many fatalities and economic losses for vulnerable and developing countries such as the Philippines. It is crucial that during calamities, on-ground surveillance is supplemented by low-cost and time-efficient methods such as satellite remote sensing. Diwata-2 is a Philippine microsatellite specifically equipped for disaster assessment. In this study, the capabilities of this satellite in ashfall detection were explored by closely examining the case of the Taal volcano eruption on January 12, 2020. Satellite images covering parts of CALABARZON and Metropolitan Manila before and after the phreatomagmatic eruption were compared. The presence and extent of heavy ash over the study area were identified after the image classification using the Support Vector Machine (SVM) algorithm. A decrease in vegetation cover and built-up areas was also observed. Upon validation, an overall accuracy of 91.4562 and Kappa coefficient of 0.8833 were achieved for the post-eruption ashfall extent map, exhibiting the potential of Diwata-2 imagery in monitoring volcanic eruptions and similar phenomena.
SUPPLEMENTING SATELLITE IMAGERY WITH SOCIAL MEDIA DATA FOR REMOTE RECONNAISSANCE: A CASE STUDY OF THE 2020 TAAL VOLCANO ERUPTION
Abstract. Social sensing and satellite imagery are named as the top emerging data sources for disaster management. There is a wealth of data, both in quantity and quality that can be extracted from social media platforms such as Twitter, given that the content published by users is generally in real-time and includes a geotag or toponym. To reduce costs, risks, and time, performing reconnaissance using remote sources of information is highly suggested. This study explores how social media data can be used to supplement satellite imagery in post-disaster remote reconnaissance using the January 2020 Taal Volcano Eruption in the Philippines. Tweets about the volcanic eruption were scraped, and ashfall-affected locations mentioned in tweet content were extracted using Named Entity Recognition (NER). To visualize the progression of the tweeted locations, dot density maps and hotspot maps were generated. Additionally, a potential ashfall extent map was generated from processed DIWATA-2 satellite imagery using Support Vector Machine (SVM) classification. An intersection of both dot density map and ashfall extent map was performed for comparative analysis of both data. Validation was carried out by matching the ashfall-affected locations with ground reports from local government offices and news reports. The use of social media data complements satellite image classification in the detection of disaster damage for a quick and cost-efficient remote reconnaissance. This information can be utilized by rescue teams for faster emergency response and relief operations during and after a disaster.
Hazardous base surges of Taal’s 2020 eruption
AbstractAfter 43 years of repose, Taal Volcano erupted on 12 January 2020 forming hazardous base surges. Using field, remote sensing (i.e. UAV and LiDAR), and numerical methods, we gathered primary data to generate well-constrained observed information on dune bedform characteristics, impact dynamic pressures and velocities of base surges. This is to advance our knowledge on this type of hazard to understand and evaluate its consequences and risks. The dilute and wet surges traveled at 50-60 ms−1 near the crater rim and decelerated before making impact on coastal communities with dynamic pressures of at least 1.7 kPa. The base surges killed more than a thousand livestock in the southeast of Taal Volcano Island, and then traveled another ~ 600 m offshore. This work is a rare document of a complete, fresh, and practically undisturbed base surge deposit, important in the study of dune deposits formed by volcanic and other processes on Earth and other planets.
The Application of Interferometric Synthetic Aperture Radar (InSar) on Damaged Area Mapping: The Case of the 2020 Taal Volcano Eruption
Geochemical and isotopic evidence of volcanic plumbing system processes from fumarolic gases of taal volcano, philippines, prior to the january 2020 eruption, factors affecting response actions of the 2020 taal volcano eruption among filipinos in luzon, philippines: a structural equation modeling approach, hazardous base surges of taal’s 2020 eruption.
Abstract After 43 years of repose, Taal Volcano erupted on 12 January 2020 forming hazardous base surges. Using field, remote sensing (i.e. UAV and LiDAR), and numerical methods, we gathered primary data to generate well-constrained empirical information on dune bedform characteristics, impact dynamic pressures and velocities of base surges to advance our knowledge on this hazard to understand and evaluate its consequences and risks. The base surges traveled at 50-60 km/hr near the crater and decelerated before making impact on coastal communities with dynamic pressures of at least 1.7-2.1 kPa. The base surges killed more than a thousand livestock in the southeast of Taal Volcano Island, and then traveled another 600 meters offshore. This work is a rare document of a complete, fresh and practically undisturbed base surge deposit, important in the study of dune deposits formed by volcanic, and other processes on Earth and other planets.
Export Citation Format
Share document.

An official website of the United States government
The .gov means it’s official. Federal government websites often end in .gov or .mil. Before sharing sensitive information, make sure you’re on a federal government site.
The site is secure. The https:// ensures that you are connecting to the official website and that any information you provide is encrypted and transmitted securely.
- Publications
- Account settings
Preview improvements coming to the PMC website in October 2024. Learn More or Try it out now .
- Advanced Search
- Journal List
- Scientific Reports

Aerosol emissions and gravity waves of Taal volcano
Jan-bai nee.
1 Aerosol Science Research Center, National Sun Yat-Sen University, No. 70 Lien-hai Rd., Kaohsiung, 80424 Taiwan, ROC
Yuan-Pin Chang
2 Department of Chemistry, National Sun Yat-Sen University, No. 70 Lien-hai Rd., Kaohsiung, 80424 Taiwan, ROC
Chia C. Wang
The Taal volcano (14.0 N, 121.0 E) in Philippines erupted in January–February 2020, with a part of aerosols drifted northward and detected by a lidar system at Kaohsiung city (22.37 N, 120.15 E), Taiwan. The aerosol observed on Feb 11 is special for its high-altitude distributions at 4–7 km with discrete structures which can be resolved into a sinusoidal oscillation of ~ 30 min period, suggesting a case of wave event caused by the eruptions. We report in this paper the gravity wave generated by the volcanic eruptions and its effects on aerosol emissions. By studying the temperature and pressure data in the Taal region using radiosonde data, we found atmospheric gravity waves with powers correlated with the optical thickness (AOD) at 550 nm measured by the Moderate Resolution Imaging Spectrometer (MODIS) satellite. This study presents the first observation of modulation of the aerosol emissions by the volcanic gravity waves and a case of coupling of dynamics and chemistry.
Introduction
Volcanic eruptions can significantly affect the environment and climate by sending large amounts of aerosols and gases into the atmosphere 1 . For example, the recent eruption of Iceland volcano Eyjafjallajökull has severely impaired the air quality and disturbed the air traffic in Europe for a few months. Additionally, volcano eruptions can also disturb the atmosphere by producing infrasound and gravity waves as shown in past eruptions of Pinatubo, Mt. St.Helen, and El Chichon 2 – 7 . For these major eruptions, pressure oscillations of amplitudes of a few millibars (mb) and periods about 200–300 s following the initial blasts have been recorded by global instruments 3 – 7 . Atmospheric waves are generated when the air is perturbed from equilibrium by external forces like volcanic eruption, convection, wind shear and so on. The propagation of these waves may be 3D with vertical height reaching as far as ionosphere 8 – 10 . Volcanic gravity waves were also observed for moderate eruptions such as the Okmok volcano 5 and Soufrière Hills Volcano 6 among others. Although there have been extensive studies of gravity waves and their effects on various parts of atmosphere, little is known about the effects of gravity waves on the volcanic aerosols.
Gravity waves are generated when the stable air is perturbed by external agents such as convection, wind shear, or volcano eruptions. Following the perturbation, the atmosphere will oscillate because of the counteraction of gravity force to restore the displacement to its stable status similar to a box-spring oscillation. Dynamics about the volcanic gravity waves has been investigated by several authors 4 – 8 . In the past, the atmospheric gravity waves are mostly observed in atmospheric parameters, such as pressure, temperature, wind field, humidity and so on 3 , 8 . A few studies addressing the gravity wave-related effects on aerosols have been reported 11 – 13 . However, gravity waves discussed in these papers are formed by wind shear or stratospheric dynamics and are unrelated with volcanic gravity waves. Recently, Baines and Sacks 13 modelled the generation of stratospheric gravity waves by volcano eruptions but not consider their effects on aerosols. Klekociuk et al. 12 reported the displacement of stratospheric aerosols by passing gravity waves of atmospheric origin. In general, the volcanic gravity waves have been extensively studied but mostly on their effects on seismic activities and upper atmospheric air 3 – 13 while their effects on aerosols is little explored.
The present study is motivated by the observations of a high lying aerosol layer at 4–7 km with a discrete structure measured by an aerosol lidar at Kaohsiung city (KS) which is about 1000 km north of Taal. This aerosol layer is special compared to normal aerosols of pollution types which are often distributed at heights below 3 km with continuous structure in time. By using back-trajectory calculations, we find this aerosol was sourced in the Taal region. To better understand this aerosol event, we investigated the eruptions of Taal volcano by combining ground and space-based data. The paper is written in the following order: the next section is about the background information of the Taal volcano and data systems used in this study which include MODIS and Merra-2 (Modern-Era Retrospective Analysis for Research and Applications, Version 2), the HYSPLIT (Hybrid Single-Particle Lagrangian Integrated Trajectory) trajectory calculations, and the lidar system. The third section is the data analysis including both the lidar and atmospheric data in the source region. The fourth part is the discussion of gravity waves related to the Taal volcano and the effects on aerosols. The last part is the conclusion of this paper.
Background information
Taal volcano.
Taal volcano (14 N,121 E) is located at Luzon Island of Philippines about 60 km south of Manila. Taal is the second most active volcanos in the Philippines with 33 historical eruptions since AD1572 with the largest eruption in AD1754 rated as volcano index (VEI) 5, compared with Pinatubo in 1991 of VEI 6 14 . Starting Jan. 12, 2020, the volcano erupted again producing plumes of hot gases, lightnings, and tephra (volcanic materials) at heights of 10–15 km according to the report of Philippine Institute of Volcanology and Seismology (PHIVOLCS) as shown in Global Volcanism Program 15 .
The 2020 eruption is investigated by using the area-averaged aerosol optical depths (AOD) at 550 nm over the Taal region as shown in Fig. 1 which is based on MODIS data and Merra-2 model. The AOD 550 nm is observed to start to rise in mid-January with the largest AOD in the end of January to early February and a few peaks in various days. More detailed introduction of MODIS and Merray-2 model are given in the following sub-sections.

Merra-2 Model aerosol extinction at 550 nm and MODIS deep blue time series, area-averaged AOT 550 nm over Taals region (in areas of Longitudes:119°–123°, Latitudes 12°–16°).
MODIS satellite
The MODIS data in Jan.–Feb. 2020 are downloaded to study the aerosol optical depth over Taal region. MODIS data are daily measurements based on observations of Terra and Aqua satellites launched by NASA in 1999 and 2002, respectively. Both satellites observe Earth using 36 bands of wavelengths ranging from 0.41 to 15 μm. This spectral diversity allows MODIS to retrieve aerosol optical parameters characterizing aerosol sizes and properties 16 – 19 . The deep blue products used in this study are designed for bright-reflecting land surfaces, such as desert, semiarid, and urban regions 19 . The MODIS aerosol optical depths (AOD) at 550 nm can be downloaded from the NASA Giovanni website.
Merra-2 model
Since most satellite observations are daily measurements based on their orbit design, the data are discrete in time. For example, Terra MODIS and Aqua MODIS are viewing the entire Earth's surface every 1 to 2 days so that the dataset are with spatial and temporal discontinuities. In general satellite measurements are also affected by cloud interferences causing breaks in cloudy days. The Merra-2 is the reanalyses of atmospheric data by combining model fields with observations of irregular space and time into a spatially complete gridded dataset. Reanalysis means a scientific method for developing a comprehensive record of atmospheric parameters. Currently, the MERRA-2 dataset spans the period 1979 through February 2016 producing data on a geographically 0.5° × 0.66° grid with 72 layers. In this work, we have used the time-series, area-averaged total aerosol scattering AOT 550 nm at Taal region. For aerosol research, MODIS provides the vast majority of AOD observations assimilated in MERRA-2 20 . For more information, please visit the NASA MERRA-2 website.
HYSPLIT system
HYSPLIT, developed by ARL (Air Resources Laboratory, NOAA), is designed to compute sample air parcel trajectories, transport, dispersion, chemical transformation, and deposition simulations 21 , 22 . Fleming et al. 21 presented a review about the development of the HYSPLIT systems for understanding the influence of air-mass history of atmospheric pollution parcels and to examine the source–receptor relationships in the timescales of long- range transport. This model involves a hybrid calculation between the Lagrangian approach and the Eulerian approach. The former uses a moving frame of reference for the traveling air parcels, the later used a fixed 3D frame to compute the paths of moving pollution.
The back-trajectory calculations in this paper are carried out by using the GDAS model (Global Data Assimilation System) with one-degree resolution (latitude-longitude) to calculate the paths of Kaohsiung air at 1000 m and 4000 m heights in the past 5 days since aerosols at both layers were observed by the lidar system. The 1000 m layer is found to be a continental aerosol transported by the Asian Northeast Monsoon system in the wintertime with sources often found in the Taklamakan or Gobi deserts. This aerosol is not further studied. Aerosols at 4000 m, at the base height in the lidar measurements, are traced to Taal region at about 2000 m heights as shown in Fig. 2 a. They were raised to 4000–7000 m heights arriving KS, Taiwan. Taal is located within the ITCZ (Inter-Tropical Convergence Zone) of ± 15° latitudes where predominant rising air exists due to strong convective systems in the tropics. Figure 2 b shows the forward trajectories starting from Taal region on Feb. 8. Based on backward studies, aerosols on Feb. 8 in the source region transported to KS, Taiwan. In order to confirm this, all forward transport paths for aerosols in the next 5 days starting Feb. 8 are calculated. Forward study shows aerosols from Taal region can reach to Taiwan area although most of them were transported to the Southeast Asia. A combination of backward and forward trajectories allows better understandings about the source of aerosols.

( a ) Five-day back-trajectories for aerosols at 1 km and 4 km layer, both were observed by lidar at Kaohsiung. The 4 km layer (blue) can be trace to Taal region 3 days ago on Feb. 8 at 2000 m while the 1 km layer (red) is traced to northwestern China from upper air. ( b ) forward trajectories from Taal region starting Feb.8 (based on backward study in a ) for all possible transport paths in the next 5 days. The results show a small part of aerosols may move to north in KS while the majority went to Southeast Asia. Figures are plotted using HYSPLIT Trajectory Model provided by ARL NOAA 21 , 22 .
Lidar systems and measurements
The lidar system used in this study is located in the campus of National Sun Yat-sen University in Kaohsiung city (22.37 N, 120.15 E). It has been setup to observe aerosols routinely since 2019. The lidar system consists of a laser transmitter using the 532 nm laser wavelength and a receiver of a 20 cm diameter Cassegrain telescope (Celestron 8) as shown in our previous publication 23 . Laser light sending to the sky will continually interact with the atmosphere with the backscattering signals collected by the telescope. Lidar signals are measured by using a combination of photomultiplier tube (Hamamatsu 9880–10), interference filters, and polarization filters. Signals are analyzed by a transient digitizer (Licel system) to generate profiles of signals with a height resolution of 3.75 m. The polarization dependent backscattering signals detected in parallel and perpendicular directions relative to the polarization of the laser emission are shown in Fig. Fig.3a,b. 3 a,b. Figure 3 c displays the backscattering coefficients (1/m-sr) derived by an inversion mechanism which has been reported previously 23 .

(left) Aerosol layers measured by lidar at Kaohsiung in signals of perpendicular ( a ) and parallel ( b ) polarization channels, time starting 18:04 LT. ( c )The backscattering coefficients in unit of (1/m-sr) measured on Feb 11.
Analyses and results
Optical properties of taal aerosols.
The optical properties of aerosols observed by lidar are discussed here. The backscattering coefficients shown in Fig. 3 c are used to derive the backscattering ratios (BR) shown in Fig. 4 a. BR is defined as ratio of backscattering coefficient relative to that of the Rayleigh scattering of air. From Fig. 4 a, we find the peak BR is at 5.78 km. The mean optical depth based on the lidar data is 0.22 ± 0.26 which may be compared with Merra-2 and MODIS AOD at 550 nm shown in Fig. 1 . The depolarization ratio (DR), defined as the ratio of signals of perpendicular to parallel polarizations, is a parameter for describing the shape of particles, with zero DR for round particles such as liquid droplets of stratospheric aerosols 1 . For the Taal case, we find a DR with a mean of 0.251 ± 0.096 at heights of 4–7.5 km as shown in Fig. 4 b, corresponding irregular shaped ashes. Volcanic aerosols are sources of stratospheric aerosols which are in the forms of droplets of sulfuric acid 1 . For the Taal eruption, the aerosols detected are likely small ashes from the eruptions just a few days ago not yet reaching stratosphere. The depolarization ratio of Taal volcano may be compared with that of Eyjafjallajökull which has a depolarization ratio about 0.35 as reported by several groups 23 – 25 .

( a ,left) Time series of integrated signals in 4.5–6.00 km heights, ( b ,right) FFT power of signals.
Gravity wave of Taal
The wave characteristics of aerosols are studied in the following ways. From Fig. Fig.3a,b, 3 a,b, we can see the aerosol are mainly distributed over 4–7 km height ranges. The peak-to peak signals over 3–8 km heights are integrated and plotted against time as shown in Fig. 5 a in which a sinusoidal oscillation of a period about 30 min lasting for about an hour is observed. The integrated signals are further analyzed by the Fast Fourier Transform (FFT) with the power spectrum showing a major peak period of 32.5 min and a minor one at 16.22 min in Fig. 5 b. The secondary peak is half of the period, or double frequency, of the major peak. We attribute this oscillation to the perturbation of aerosols by gravity waves. It is likely that the eruption of the Taal volcano perturbed the emissions of aerosols, producing discrete distributions of aerosol parcels that eventually transported to distant regions as observed by the lidar system in KS.

( a , left) Time series of integrated signals in 3–8 km heights ( b , right) FFT power of signal of Fig. (5a) with the major peak at 32.5 min and a minor peak at 16.2 min.
To understand the atmospheric conditions in the source region, we have studied the temperature profiles of the Taal region by using the radiosonde data (Station no. 98443, Lat. 14.56, Long. 121.36) for two months of January–February 2020. The temperature data of several days corresponding to before (Jan 1) and during (Feb.1 and 8) the eruptions are shown in Fig. 6 a. The variations of temperature (or pressure, not presented here) for these days are investigated in the following ways. We first derived the mean temperature profiles of each day by using a polynomial fitting technique. The temperature perturbations for these days are then calculated by subtracting the mean temperatures from the observed temperature profiles as shown in Fig. 6 b. The Lomb–Scargle periodogram (LS) which is suitable for discrete data analysis is applied to find the characteristic frequencies of oscillations using the temperature anomaly in Fig. 6 b. The LS results in Fig. 7 show atmospheric waves of vertical wavelengths of 3–5 km in heights of 1–10 km. Results of Fig. 7 is typical for finding gravity wave activities in the atmosphere.

( a ) Temperature profiles of Taal region on Jan 1, Feb 1, and Feb 8; ( b ) Temperature anomalies.

Power spectrum of the Lomb-Scargle analyses for temperature anomaly of Fig. 6 b.
In the power spectra of Fig. 7 , the strongest peak appears on Feb.1 whose AOD 550 nm is also the largest as shown in Fig. 1 . The smallest power intensity occurs on Jan. 1 corresponding to the day of before the eruption, and a moderate power for Feb. 8 during the eruption. Feb. 8 is the origin of lidar aerosols based on back-trajectory studies discussed earlier. Therefore, the powers of the wave activities correlate well with the optical thickness of the region given by MODIS AOD and Merra-2 model. Similar results are also found for pressure data but with much lower powers and are not shown here. Table Table1 1 lists the relevant parameters discussed above.
A list of parameters.
Here we briefly describe the gravity waves to gain insight into the possible perturbations produced by the Taal eruptions. The displacement of air in a stable atmosphere is explained by the Brunt-Vaisala (BV) frequency, N(z), which is the frequency of air parcel oscillation. The frequency N(z) is calculated by the following formula
where g is the gravity constant 9.8 m/s 2 , c p is heat capacity of air 1004 J/deg-kg, and T(z) is the temperature. N(z) is found to be 0.022 rad s −1 at z = 5 km, corresponding to a period about 280 s which is close to ~ 300 s period described in earlier studies about volcanic gravity waves 4 . The BV frequency is the maximum possible frequency for the gravity wave since the wave can propagate both horizontally and vertically 8 . Considering only 2D plane wave for simplicity, the wave function can be written as
where u’ is the perturbation of the atmospheric parameters including temperature, wind speed etc., k and m are the horizontal and vertical wavenumbers, ω is the wave frequency. The relationship between frequency and wavenumber is given by the dispersion relation 2 , 8
where N is the Brunt–Vaisala frequency, and f is the Coriolis inertial frequency of the earth rotation at a latitude α defined as f = 2Ωsin α = 3.51 × 10 –5 s -1 , Ω is the earth’s rotation speed. For a 2D wave propagating at a directional angle β relative to the horizontal direction, the frequency is:
If there is a background horizontal wind of speed u o , the wave frequency is modified by the wind vector u o as 8
Although the vertical wavelength is a few kilometers as shown in Fig. 7 , the horizontal wavelength may be hundreds or thousands of kilometers 8 . The volcanic gravity waves can modulate the aerosols to cause oscillations but with a frequency different from that of air because aerosols are heavier than air. The average aerosol mass is about 1.5 g/cm 3 24 – 26 which is 1000 times or more than the density of air. Using a relationship ω ~ 1/m -2 based on box spring oscillation, we find aerosols should have a wave period about 2000s by comparison with the BV frequency of 280 s of air. This estimation is close to the lidar observations but may be fortuitous. The gravity wave parameters discussed above are summarized in Table Table1 1 .
From Fig. Fig.3a,b, 3 a,b, we found that aerosols are mainly distributed over heights of 4–7 km. This vertical spread should result from the action of oscillations produced by the gravity waves and can be understood in terms of the box-spring model. At the spring’s maximum stretch, aerosols are pushed to the peak height over 7 km and back to the lowest height when the spring is relaxed. Therefore, gravity waves perturbed the distribution of aerosols vertically with a structure related with the oscillation period.
Recently Klekociuk et al. 12 reported a case of stratospheric aerosols modulated by the gravity wave. A displacement of stratospheric aerosols over a distance of 600 m was observed produced by passing gravity waves. The perturbation will cause aerosols to distribute over different heights or different atmospheric temperatures which can affect the condensation or coagulation of aerosols. In the present case, the spread is much broader and the temperature variation is about 10 degrees which can significantly affect aerosol condensation, coagulation, and cloud formation. Furthermore, variation of the coagulation of aerosols also affects the sedimentation of aerosols that can impact the environment. These effects remain to be further clarified but is beyond the scope of this paper.
Volcanic aerosols have long been studied to better understand their roles in affecting the environment and climate of Earth. However, volcanic eruptions can also induce dynamic activities of the atmosphere by producing gravity waves which may influence the distributions of gas or aerosol emissions. It is well known that volcanic emissions of CO 2 , H 2 O, and SO 2 , in addition to aerosols, are important in affecting the stratospheric chemistry and greenhouse effects. However, their dynamic effects have not been studied. In this paper, we report a case of a discrete aerosol layer produced by Taal eruption observed by a lidar system at Kaohsiung city, Taiwan, which is 1000 km north to the Taal region. The integrated signals over 3–8 km show a sinusoidal oscillation with a period about 32.5 min. With additional data of MODIS AOD, Merra-2 model, radiosonde temperature, and back-trajectory calculations, we studied the possible perturbation of volcanic aerosols by the volcanic gravity waves. The temperatures profiles from the radiosonde data of the source region are analysed by Lomb-Scargle (LS) periodogram for days before and during the eruptions for understanding the atmospheric dynamics with the power spectra showing correlation with the AOD of these days. These results support the possibility of gravity waves generated by the volcanic eruptions can affect aerosol emissions producing aerosol layers distributed over different heights when transport to distant regions. However, considering the long transport for aerosols to reach the lidar site, we cannot rule out possibilities of the gravity wave activities also caused by the convective clouds or other weather systems in the tropical region where aerosols travelled, although such events have not been observed by us so far. Under either condition, this is a case of modulation of aerosol structures by atmospheric dynamics resulting redistribution of aerosols spatially with the possibility of affecting the coagulation, condensation, sedimentation, and cloud formation. These effects have so far not been systematically explored but is noteworthy for future research in the context of aerosol-climate systems.
Acknowledgements
This research is supported by National Sun Yat-sen University (NSYSU) and Ministry of Education. Figure Figure2 2 was plotted with HYSPLIT model which is provided by the National Oceanic and Atmospheric Administration (NOAA) Air Research Lab. 21,22 Radiosonde data of Station no. 98443 is obtained from the University of Wyoming, Department of Atmospheric Science. The MERRA-2 data used in this study is provided by the Global Modeling and Assimilation Office (GMAO) at NASA Goddard Space Flight Center. MODIS AOD and Merra-2 model are provided by NASA Giovanni network. Graduate students Bo Kai Su and Jou-Yun Chen had participated the lidar operation for this observation. Finally, the reviewers are acknowledged for their comments which greatly improved the manuscript.
Author contributions
J.B.N. setup lidar system, analyzed data, write and edit paper. Y.P.C. setup lidar, help observations, edit paper, C.C.W., project management and edit paper.
Aerosol Science Research Center, National Sun Yat-sen University; Higher Education Sprout Project of the Ministry of Education, Ministry of Science and Technology, Taiwan, R. O. C. (Projects No. MOST107-2113-M-110-004-MY3, MOST109-2113-M-110-010 and MOST110-2113-M-110-019).
Competing interests
The authors declare no competing interests.
Publisher's note
Springer Nature remains neutral with regard to jurisdictional claims in published maps and institutional affiliations.

COMMENTS
1 Introduction. Taal volcano is a caldera located in southwestern Luzon island in the Philippines (Figure 1).It is one of the frequently erupting volcanoes in the country, having at least 33 known historical eruptions between CE1572 and CE1977 (Delos Reyes et al., 2018).The volcano is part of the Macolod Corridor, a complex NE-SW trending 50-60 km-wide rift zone, characterized by active ...
Taal Volcano is an active volcano located in Batangas Province, Luzon, Philippines (Fig. 1).It is a 311-m high stratovolcano situated in the center of a Taal lake [1].It is also known to be one of the 15 most dangerous "Decade Volcanoes", imposing high hazardous risks among its nearby population centers [2] due to its proximity.In addition, Taal Volcano ranks as the second most active out ...
A comprehensive review of the historical eruptions of Taal Volcano is the central element of this paper and includes all eruptions from AD1572 (the first known historic event) to AD1977. Eruption styles and the interplay between processes and products for each eruption are reinterpreted based on the narrative descriptions from all available ...
In addition, Taal Volcano ranks as the second most active out of the 24 active volcanoes in the country with 33 recorded eruptions since its first documented eruption in the year 1572 [3]. On January 12th, 2020, the Taal volcano erupted (Fig. 2). The Philippine Institute of Volcanology and Seismology (PHILVOCS) issued an Alert Level 4 status[26].
This study reconstructs the 12 January 2020 Taal Volcano eruption through the analysis of narratives from two perspectives: those of the Taal Volcano Island (TVI) residents and those living along the Taal Caldera Lakeshore (TCLS). Personal accounts of TVI residents provide an up-close look at the volcano's behavior from the day before the eruption to the escalation of volcanic activity until ...
Taal volcano has produced 33 historical eruptions, and five notable eruptions generated tsunamis in the lake in 1716, 1749, 1754, 1911 and 1965 [3, 4]. The 1716 eruption was a subaqueous volcanic ...
After 43 years of dormancy, Taal Volcano violently erupted in January 2020 forming a towering eruption plume. The fall deposits covered an area of 8605 km2, which includes Metro Manila of the National Capital Region of the Philippines. The tephra fall caused damage to crops, traffic congestion, roof collapse, and changes in air quality in the affected areas. In a tropical region where heavy ...
The Taal volcano (14.0 N, 121.0 E) in Philippines erupted in January-February 2020, with a part of aerosols drifted northward and detected by a lidar system at Kaohsiung city (22.37 N, 120.15 E ...
Taal volcano is in a caldera system located in southern Luzon island and is one of the most active volcanoes in the Philippines. It has produced around 35 recorded eruptions since 3,580 BCE, ranging from VEI 1 to 6, with the majority of eruptions being a VEI 2. The caldera contains a lake with an island that also contains a lake within the Main ...
Merra-2 (Modern-Era Retrospective Analysis for Research and Applications, Version 2), the HYSPLIT (Hybrid ... of this paper. Background information Taal volcano. Taal volcano (14 N,121 E) is ...
Geophysical Research Letters is an AGU journal publishing high-impact, innovative articles on major advances spanning all of the major geoscience disciplines. Abstract On January 12, 2020, Taal volcano, Philippines, erupted after 43 years of repose, affecting more than 500,000 people.
Taal volcano (311 m in altitude) is located in The Philippines (14°N, 121°E) and since 1572 has erupted 33 times, causing more than 2,000 casualties during the most violent eruptions. In March 2010, …
On January 12, 2020, Taal volcano, Philippines, erupted after 43 years of repose, affecting more than 500,000 people. Using interferometric synthetic aperture radar (InSAR) data, we present the pre‐ to post‐eruption analyses of the deformation of Taal. We find that: (1) prior to eruption, the volcano experienced long‐term deflation followed by short‐term inflation, reflecting the ...
Map of sulfur dioxide (SO2) emitted by the Taal volcano on June 29, 2021, as observed by the NASA/NOAA Suomi NPP satellite. Credits: NASA. The NASA Earth Applied Sciences Disasters program area has activated efforts to monitor Taal Volcano with Earth-observing data for air quality concerns, surface deformation, or any signs that could indicate the potential for a larger eruption.
A comprehensive review of the historical eruptions of Taal Volcano is the central element of this paper and includes all eruptions from AD1572 (the first known historic event) to AD1977. Eruption styles and the interplay between processes and products for each eruption are reinterpreted based on the narrative descriptions from all available ...
Abstract. The city of Metro Manila has been constantly battered by several hazards on an annual basis. On January 2020, the Taal Volcano erupted with multiple recorded earthquakes. Previous literatures have found that Metro Manila is experiencing a steady subsidence. Determination of land uplift or subsidence is crucial in planning and ...
A comprehensive review of the historical eruptions of Taal Volcano is the central element of this paper and includes all eruptions from AD1572 (the first known historic event) to AD1977.
The recent eruption of Taal volcano and Mt. Bulusan in the Philippines affected several people due to the lack of resources, awareness, and preparedness activities. ... Discover the world's ...
The 2020 Taal Volcano eruption resulted in one reported dead and one missing (Cinco, 2020). It was unlike the previous eruptions of Taal Volcano, such as those in the 1911 and 1965 eruptions, where there were many fatalities. The 12 January 2020 Taal volcano eruption is considered a test to the Philippines' disaster preparedness (Minter, 2020).
Volcanic eruption activity across the world has been increasing. The recent eruption of Taal volcano and Mt. Bulusan in the Philippines affected several people due to the lack of resources, awareness, and preparedness activities. Volcanic eruption disrupts the sustainability of a community. This study assessed people's preparedness for volcanic eruption using a machine learning ensemble ...
The paper is written in the following order: the next section is about the background information of the Taal volcano and data systems used in this study which include MODIS and Merra-2 (Modern-Era Retrospective Analysis for Research and Applications, Version 2), the HYSPLIT (Hybrid Single-Particle Lagrangian Integrated Trajectory) trajectory ...
Hence, this study analyzed the media coverage of the 2020 Taal Volcano Eruption vis-à-vis the framework of disaster journalism and using the lenses of the Framing Theory and Social Amplification of Risk Theory. A total of 384 stories from 6 nationally-circulated newspapers were sampled.
RESEARCH PAPER | Philippine Journal of Systematic Biology ... The Taal Volcano Protected Landscape (TVPL) is known as a prehistoric volcanic caldera. It was postulated from
A long-term deflation of the Taal Caldera as well as a short-term inflation of the general northern and southeastern flanks of the Taal Volcano Island were also observed. On April 25, 3,383 tonnes of sulfur dioxide emitted from the volcano amid the upwelling of hot volcanic fluids in the Main Crater Lake.