- Reference Manager
- Simple TEXT file

People also looked at
Specialty grand challenge article, the immunology of parasite infections: grand challenges.
- School of Life Sciences, University of Technology Sydney, Sydney, NSW, Australia
Introduction
Parasitic diseases continue to be a major cause of morbidity and mortality worldwide, with billions of people at risk of infection, often with multiple parasites ( https://www.who.int ). Despite this global impact, there are limited treatment options for most infections and only one human vaccine, which is moderately effective against malaria ( Stutzer et al., 2018 ; Zavala, 2022 ). The key to controlling parasite infection is to better understand the host immune response to these pathogens and to determine how this can be exploited to protect the host from infection and/or the pathological consequences of infection. However, this is a formidable task. By virtue of a dependency on a host for cyclical transmission and millennia of co-evolution with their hosts, parasites have developed multifaceted, often life-cycle stage-specific strategies to utilize, evade, modulate, and/or regulate the host immune response to avoid elimination and ensure their long-term survival to mature and complete their life cycle ( Yazdanbakhsh and Sacks, 2010 ).
Through decades of research many of these mechanisms have been characterized ( Chulanetra and Chaicumpa, 2021 ), and can be subdivided into different tactical approaches including, an ability to become camouflaged by host factors, inhabiting immune privileged sites, expressing stage specific antigens, secreting components that mimic host factors, driving the differentiation of immune cell phenotypes, and regulating the responsiveness of host immune cells. Importantly, these studies have provided critical insights into fundamental mechanisms of human immunology. Most notably, murine experimental leishmaniasis was the first model to confirm the biological significance of the Th1/Th2 paradigm in vivo ( Scott, 1989 ). While these discoveries have significantly contributed to our current knowledge of the interaction between parasites and their host’s immune response, there is still an incomplete understanding of this relationship. Future research will be informed by concomitant developments in immunological, molecular and cell culturing techniques and innovations, such as immune-metabolomics, cell signaling systems, imaging mass spectrometry, organoid culture, microfluidics, vaccine technology (and others). However, there are also important considerations that are specific to parasite infection, and I believe represent some of the grand challenges facing this field of parasitology.
Grand challenge 1: Are parasite infections a necessary evil – is there a future for parasite derived therapeutics?
While parasites have clearly benefited from the development of immune-modulatory mechanisms, this evolutionary adaptation to support their long-term survival may have unwittingly positioned them as a necessary component of the human immune response ( Fumagalli et al., 2009 ; Jackson et al., 2009 ). Due to their ubiquitous nature in human populations, it has been proposed that their presence provided the regulatory networks to keep activation of immune responses in check and drive the homeostatic resolution of inflammation. As a result of this co-dependence, the removal of parasites from human populations through increased sanitation and urbanization establishes an imbalance in the immune system, thereby increasing susceptibility to the inappropriate activation of inflammatory responses, resulting in immune-mediated disease ( Jackson et al., 2009 ; Rook, 2010 ; Allen and Maizels, 2011 ). Indeed, there is compelling epidemiological evidence of an inverse correlation between the prevalence of endemic helminth infections and the incidence of immune-mediated disease in human populations worldwide. Furthermore, there is now a substantial body of experimental evidence in animal models showing that live parasite infection, or the administration of parasite-derived proteins/peptides, can prevent progression of inflammatory disease ( Maizels, 2020 ; Ryan et al., 2020 ). Understandably, much of this research has been performed from the perspective of parasitology research, rather than a translational, clinical pathway. However, this means that the most appropriate pre-clinical models of immune-mediated disease have not always been utilized and that fundamental knowledge of the pharmacokinetics, mechanisms of action, and toxicity of the parasite molecules is often lacking ( Sobotková et al., 2019 ).
The challenge here will be the establishment of a concerted, collaborative effort between research and industry partnerships. This will more effectively inform research activity to better position parasite-derived therapeutics as a consideration for clinical applications by the pharmaceutical industry and drive this solid (and encouraging) evidence of efficacy from bench to bedside.
Grand challenge 2: Parasites do not live alone – a holistic approach is needed
In the same way the modulation of immune responses by parasites may impact the course of immune-mediated diseases, parasites can also significantly alter the developing immune response to concurrent infections, which consequently impacts the progression and severity of illness, and vice versa ( Hassan and Blanchard, 2022 ). This is a particular concern for endemic human populations (and most animals), in which parasite infections occur against a background of co-infection with other parasites and other microbial pathogens ( Venter et al., 2022 ). However, there is no clear consensus as to which concurrent infections are protective or which increase the severity of disease, nor is there a clear understanding on how the presence of multiple pathogens specifically influences the host immune response ( Mabbott, 2018 ). Despite the important consequences of co-infection, the potential interactions among parasites are rarely considered in either clinical settings or in immunological analysis of models of infection. However, understanding how the host immune response behaves in this setting is critical to informing possible strategies for treatment and/or infection control.
In addition to the presence of pathogenic organisms, trillions of commensal microorganisms, inhabit the mammalian body. This microbiota underpins the health of its host with hypothesized impacts on inflammation, immune development, and disease outcomes. Evidence is emerging that the host microbiome plays a contributory role in the parasite’s modulation of host immune responses. Multiple studies have now shown that the presence of parasites in the intestine changes the overall composition of bacterial species in the gut ( Zaiss and Harris, 2016 ; Chabé et al., 2017 ), which in turn influences inflammation and health ( Ramanan et al., 2016 ; Coakley and Harris, 2019 ). Whether the host immune response to the parasite infection is altering the resident microbiota, and/or whether the parasites and their secreted products are directly modifying the composition of the microbiome remains to be elucidated.
In addition to the host microbiota, parasite-associated microbiota also shapes the host immune response and the pathological outcome to disease ( Ives et al., 2011 ; Yurchenko and Lukeš, 2018 ). However, despite the identification of the Wolbachia endosymbiont of filarial nematodes more than 20 years ago ( Williams et al., 2000 ), only a handful of parasite species have had their associated microbiome characterized ( Dheilly et al., 2019 ). As a result, there is limited insight into the functional relationship between the parasite, its microbiome and the host and the consequences that this has upon the immune response and the outcome to infection.
This evidence of the involvement of microorganisms in the parasite-host relationship is a reminder of the complexity of a mammalian biological community. Disentangling the interactions between parasites and hosts, their respective microbiota, and microbial pathogens in the parasite’s direct environment, and understanding how these influence the host immune response will be a challenge. A holistic approach is required, accounting for all contributors, to elucidate the critical determinants of risk and benefit to host health.
Grand challenge 3: The importance of the host – is the lab mouse an adequate model?
The majority of research elucidating the fundamental mechanisms of parasite immune modulation/evasion, has been performed in experimental animals, particularly rodents. This methodology has been driven by the broad availability of different strains (and transgenics) of mice and a multitude of murine specific reagents to support the characterization of immunological networks and cellular phenotypes. Although this approach has been central to the identification and characterization of immune responses during infection, it has likely only illuminated a small fraction of the heterogeneity in host-parasite immune relationships.
While laboratory mice have served well as models of mammalian immune function, generating many successful translational therapies to the clinic, it has been now well established that the immune cell populations in these mice are not equivalent to adult mammals and thus do not always exhibit corresponding immune responses to infection ( Abolins et al., 2017 ; Masopust et al., 2017 ). The phenotype and functional activity of an animal’s immune response to infections is informed by their environment. In the laboratory setting, mice are generally housed and maintained in filtered, microbial free, controlled environments which is a contributing factor to the immunological divergence between lab mice and wild mice (and other mammals) ( Graham, 2021 ), a difference which has been shown to influence the outcome of parasite infection. While the lab strain of C57BL/6 mice are resistant to high infectious doses of the intestinal nematode, Trichuris muris , when these mice were exposed to outdoor environments, they displayed a reduced capacity to produce Th2 type immune responses and as a result their susceptibility to infection was significantly increased ( Leung et al., 2018 ). The factors that contributed to this change in immune response were not determined, but it is a scenario that should be extended to the study of other parasite infections.
Despite the vast number of identified helminths and protozoan parasites ( Cox, 2002 ), our assumptions regarding the immune response to parasite infection has largely been formed from studies of only a handful of parasites that have the capacity to infect a laboratory mouse. Furthermore, the primary mechanisms of immune modulation attributed to helminths has generally been elucidated through infections with nematodes that have only adapted to infect a rodent host ( Heligmosomoides polygyrus , Trichuris muris). While these may have been proven to be an adequate model for human intestinal helminth infection, there is no equivalent murine model for many of the larger tissue dwelling helminth parasites from which to elucidate immune modulatory mechanisms throughout their life cycle, as the tissue damage that ensues during maturation and migration of the adult parasites in these cases is often too severe for a murine host. For these parasites, large animal hosts are required to fully understand the host-parasite immune relationship, but the logistics, ethics, cost, and lack of suitable analytical reagents is often prohibitive.
To establish an infection in these models (mouse and large animal), in general, a single large infectious dose is administered to the host and the immune response and corresponding pathogenesis is tracked over time. Once again, although this is a necessary approach to gain some insight into the developing immune response to the presence (and development) of a pathogen, it is unlikely to represent a naturally occurring infectious dose. As such, it is important to consider how the pathology resulting from a high parasite burden is impacting the developing immune response and determine whether this is producing an artificial representation of the true parasite-host relationship. In addition, this experimental protocol of infection may be negatively impacting the evaluation of vaccine candidates. The potent immune modulation induced by such high infectious doses of parasites likely suppresses the antigen-specific responses induced by vaccination. In contrast, a low dose (or trickle dose in field trials for animal hosts) would better represent the real-world challenge for a vaccine.
Combined, these observations highlight the challenge in selecting models of parasite infection that authentically reflect that of their natural hosts and therefore accommodate the characterization of the true phenotype and functional activity of the host immune response during all stage of infection.
Grand challenge 4: Expanding the characterization of parasite-host communication
A critical aspect to uncovering the mechanisms by which parasites control their host’s immune response is to characterize the communication signals used by the parasite. Accordingly, over several decades the excretory/secretory (ES) products of many parasites have been characterized. Starting with the identification of the proteins released by the parasites, the development of modern techniques enabled more in-depth characterization and expanded the repertoire of secreted molecules to glycans, lipids, miRNAs, small molecules and metabolites ( Maizels et al., 2018 ; Ryan et al., 2022 ). Many of these components are homologous to host molecules involved in the regulation of immunity, thus endowing them with the capacity to mimic host signaling networks to modulate the immune response ( Smyth et al., 2018 , Ricafrente et al., 2021 ). Furthermore, some of these parasite derived molecules are secreted by the parasite within extracellular vesicles (EV), which are taken up by host cells ( Wu et al., 2019 ), thus creating a cross-species channel of communication and facilitating the parasites with access to the intracellular machinery of host immune cells. A greater understanding of the biogenesis of parasite EVs and the selection and sorting of their cargo will transform our understanding of the parasite-host relationship, build on the existing knowledge of mechanisms of parasite immune modulation, and provide new targets for anti-helminthic strategies.
Grand challenge 5: The characterization of a protective immune response - development of an effective vaccine
Ultimately, the study of parasite immunology is driven by the ambition of identifying effector mechanisms to eliminate the parasite and prevent future infections. However, despite the extensive characterization of the parasite’s mechanisms of immune modulation and immune evasion, the ability and capacity to therapeutically intervene in these infections remains rudimentary. Fundamentally, development of an effective parasite vaccine remains hampered by a lack of understanding of what constitutes a protective immune response. Although multiple clinical trials have suggested efficacy of putative vaccine candidates, most have not translated to the field.
Perhaps it is time to challenge thinking and the approach to parasite vaccine development. One question that needs to be asked is how good a vaccine against any specific parasite needs to be? After all, the only human parasite vaccine on the market is estimated to provide just a short-lived protection of ~34% ( Zavala, 2022 ), an efficacy that would not be accepted by any standard vaccine development program. However, by reducing severe disease in young children, and when administered in conjunction with other control measures it may decrease exposure to the parasite, and therefore the vaccine will contribute to minimize or eliminate the clinical consequences of infection. So rather than trying to achieve resistance, perhaps the goal should be reimagined with the aim of minimizing or eliminating the pathological consequences of infection.
Invitation to the immunology and immune evasion section of frontiers in parasitology
These grand challenges are not an exhaustive list, and as such The Frontiers in Parasitology Immunology and Immune Evasion Section is calling on the research community to initiate further conversation, to inspire and to provide informed guidance regarding the advances and research questions that are most important to them. Accordingly, we have released a call for reviews covering any topic in Parasite Immunology and Immune Evasion and currently have two open Research Topics named “Insights into Recent Advances and Future Perspectives in Parasite Immunology” and “Helminth Infection and Regulation of Unrelated Diseases”. We look forward publishing experimental work, clinical trials, perspectives, opinions, and reviews in this field of research.
Author contributions
The author confirms being the sole contributor of this work and has approved it for publication.
Conflict of interest
The author declares that the research was conducted in the absence of any commercial or financial relationships that could be construed as a potential conflict of interest.
Publisher’s note
All claims expressed in this article are solely those of the authors and do not necessarily represent those of their affiliated organizations, or those of the publisher, the editors and the reviewers. Any product that may be evaluated in this article, or claim that may be made by its manufacturer, is not guaranteed or endorsed by the publisher.
Abolins S., King E. C., Lazarou L., Weldon L., Hughes L., Drescher P., et al. (2017). The comparative immunology of wild and laboratory mice, mus musculus domesticus. Nat. Commun. 8, 14811. doi: 10.1038/ncomms14811
PubMed Abstract | CrossRef Full Text | Google Scholar
Allen J. E., Maizels R. M. (2011). Diversity and dialogue in immunity to helminths. Nat. Rev. Immunol. 11, 375–388. doi: 10.1038/nri2992
Chabé M., Lokmer A., Ségurel L. (2017). Gut Protozoa: Friends or foes of the human gut microbiota? Trends Parasitol. 33, 925–934. doi: 10.1016/j.pt.2017.08.005
Chulanetra M., Chaicumpa W. (2021). Revisiting the mechanisms of immune evasion employed by human parasites. Front. Cell Infect. Microbiol. 11, 702125. doi: 10.3389/fcimb.2021.702125
Coakley G., Harris N. L. (2019). Helminths and microbiota - partners in arthritis prevention? Nat. Rev. Rheumatol. 15, 454–455. doi: 10.1038/s41584-019-0253-3
Cox F. E. (2002). History of human parasitology. Clin.Microbiol. Rev. 15, 595–612. doi: 10.1128/CMR.15.4.595-612.2002
Dheilly N. M., Martínez Martínez J., Rosario K., Brindley P. J., Fichorova R. N., Kaye J. Z., et al. (2019). Parasite microbiome project: Grand challenges. PLos Pathog. 15, e1008028. doi: 10.1371/journal.ppat.1008028
Fumagalli M., Pozzoli U., Cagliani R., Comi G. P., Clerici S., Cagliani M., et al. (2009). Parasites represent a major selective force for interleukin genes and shape the genetic predisposition to autoimmune conditions. J. Exp. Med. 206, 395–1408. doi: 10.1084/jem.20082779
CrossRef Full Text | Google Scholar
Graham A. L. (2021). Naturalizing mouse models for immunology. Nat. Immunol. 22, 111–117. doi: 10.1038/s41590-020-00857-2
Hassan A., Blanchard N. (2022). Microbial (co)infections: Powerful immune influencers. PLos Pathog. 18, e1010212. doi: 10.1371/journal.ppat.1010212
Ives A., Ronet C., Prevel F., Ruzzante G., Fuertes-Marraco S., Schutz F., et al. (2011). Leishmania RNA virus controls the severity of mucocutaneous leishmaniasis. Science 331 (6018), 775–778. doi: 10.1126/science.1199326
Jackson J. A., Friberg I. M., Little S., Bradley J. E. (2009). Review series on helminths, immune modulation and the hygiene hypothesis: immunity against helminths and immunological phenomena in modern human populations: coevolutionary legacies? Immunology 126, 18–27. doi: 10.1111/j.1365-2567.2008.03010.x
Leung J. M., Budischak S. A., Chung The H., Hansen C., Bowcutt R., Neill R., et al. (2018). Rapid environmental effects on gut nematode susceptibility in rewilded mice. PLos Biol. 16, e2004108. doi: 10.1371/journal.pbio.2004108
Mabbott N. A. (2018). The influence of parasite infections on host immunity to Co-infection with other pathogens. Front. Immunol. 9. doi: 10.3389/fimmu.2018.02579
Maizels R. M. (2020). Regulation of immunity and allergy by helminth parasites. Allergy. 75, 524 534. doi: 10.1111/all.13944
Maizels R. M., Smits H. H., McSorley H. J. (2018). Modulation of host immunity by helminths: The expanding repertoire of parasite effector molecules. Immunity. 49, 801–818. doi: 10.1016/j.immuni.2018.10.016
Masopust D., Sivula C. P., Jameson S. C. (2017). Of mice, dirty mice, and men: Using mice to understand human immunology. J. Immunol. 199, 383–388. doi: 10.4049/jimmunol.1700453
Ramanan D., Bowcutt R., Lee S. C., Tang M. S., Kurtz Z. D., Ding Y., et al. (2016). Helminth infection promotes colonization resistance via type 2 immunity. Science 352, 608–612. doi: 10.1126/science.aaf3229
Ricafrente A., Nguyen H., Tran N., Donnelly S. (2021). An evaluation of the fasciola hepatica miRnome predicts a targeted regulation of mammalian innate immune responses. Front. Immunol. 11, 608686. doi: 10.3389/fimmu.2020.608686
Rook G. A. (2010). 99th dahlem conference on infection, inflammation and chronic inflammatory disorders: darwinian medicine and the 'hygiene' or 'old friends' hypothesis. Clin. Exp. Immunol. 160, 70–79. doi: 10.1111/j.1365-2249.2010.04133.x
Ryan S. M., Eichenberger R. M., Ruscher R., Giacomin P. R., Loukas A. (2020). Harnessing helminth-driven immunoregulation in the search for novel therapeutic modalities. PLos Pathog. 16, e1008508. doi: 10.1371/journal.ppat.1008508
Ryan S. M., Ruscher R., Johnston W. A., Pickering D. A., Kennedy M. W., Smith B. O., et al. (2022). Novel anti-inflammatory biologics shaped by parasite-host coevolution. Proc. Natl. Acad. Sci. U. S. A. 119, e2202795119. doi: 10.1073/pnas.2202795119
Scott P. (1989). The role of TH1 and TH2 cells in experimental cutaneous leishmaniasis. Exp. Parasitol. 68, 369–372. doi: 10.1016/0014-4894(89)90120-3
Smyth D. J., Harcus Y., White M., Gregory W. F., Nahler J., Stephens I., et al. (2018). TGF-β mimic proteins form an extended gene family in the murine parasite heligmosomoides polygyrus. Int. J. Parasitol. 48, 379–385. doi: 10.1016/j.ijpara.2017.12.004
Sobotková K., Parker W., Levá J., Růžková J., Lukeš J., Jirků Pomajbíková K. (2019). Helminth therapy - from the parasite perspective. Trends Parasitol. 35, 501–515. doi: 10.1016/j.pt.2019.04.009
Stutzer C., Richards S. A., Ferreira M., Baron S., Maritz-Olivier C. (2018). Metazoan parasite vaccines: Present status and future prospects. Front. Cell Infect. Microbiol. 8. doi: 10.3389/fcimb.2018.00067
Venter F., Matthews K. R., Silvester E. (2022). Parasite co-infection: an ecological, molecular and experimental perspective. Proc. Biol. Sci. 289, 20212155. doi: 10.1098/rspb.2021.2155
Williams S. A., Lizotte-Waniewski M. R., Foster J., Guiliano D., Daub J., Scott A. L., et al. (2000). The filarial genome project: analysis of the nuclear, mitochondrial and endosymbiont genomes of brugia malayi. Int. J. Parasitol. 30 (4), 411–419. doi: 10.1016/s0020-7519(00)00014-x
Wu Z., Wang L., Li J., Wang L., Wu Z., Sun X. (2019). Extracellular vesicle-mediated communication within host-parasite interactions. Front. Immunol. 9. doi: 10.3389/fimmu.2018.03066
Yazdanbakhsh M., Sacks D. L. (2010). Why does immunity to parasites take so long to develop? Nat. Rev. Immunol. 10, 80–81. doi: 10.1038/nri2673
Yurchenko V., Lukeš J. (2018). Parasites and their (endo)symbiotic microbes. Parasitology. 145, 1261–1264. doi: 10.1017/S0031182018001257
Zaiss M. M., Harris N. L. (2016). Interactions between the intestinal microbiome and helminth parasites. Parasite Immunol. 38, 5–11. doi: 10.1111/pim.12274
Zavala F. (2022). RTS,S: the first malaria vaccine. J. Clin. Invest. 132, e156588. doi: 10.1172/JCI156588
Keywords: parasite, immunology, immune-modulation, microbiota, helminth-therapy, vaccine
Citation: Donnelly S (2022) The immunology of parasite infections: Grand challenges. Front. Parasitol. 1:1069205. doi: 10.3389/fpara.2022.1069205
Received: 13 October 2022; Accepted: 25 October 2022; Published: 08 November 2022.
Edited and Reviewed by:
Copyright © 2022 Donnelly. This is an open-access article distributed under the terms of the Creative Commons Attribution License (CC BY) . The use, distribution or reproduction in other forums is permitted, provided the original author(s) and the copyright owner(s) are credited and that the original publication in this journal is cited, in accordance with accepted academic practice. No use, distribution or reproduction is permitted which does not comply with these terms.
*Correspondence: Sheila Donnelly, [email protected]
- Open access
- Published: 15 June 2019
Investigating immune responses to parasites using transgenesis
- Mebrahtu G. Tedla ORCID: orcid.org/0000-0002-0903-3269 1 ,
- Alison L. Every 1 nAff2 &
- Jean-Pierre Y. Scheerlinck 1
Parasites & Vectors volume 12 , Article number: 303 ( 2019 ) Cite this article
9617 Accesses
5 Citations
5 Altmetric
Metrics details
Parasites comprise diverse and complex organisms, which substantially impact human and animal health. Most parasites have complex life-cycles, and by virtue of co-evolution have developed multifaceted, often life-cycle stage-specific relationships with the immune system of their hosts. The complexity in the biology of many parasites often limits our knowledge of parasite-specific immune responses, to in vitro studies only. The relatively recent development of methods to stably manipulate the genetic make-up of many parasites has allowed a better understanding of host-parasite interactions, particularly in vivo . In this regard, the use of transgenic parasites can facilitate the study of immunomodulatory mechanisms under in vivo conditions. Therefore, in this review, we specifically highlighted the current developments in the use of transgenic parasites to unravel the host’s immune response to different life-cycle stages of some key parasite species such as Leishmania , Schistosoma , Toxoplasma , Plasmodium and Trypanosome and to some degree, the use of transgenic nematode parasites is also briefly discussed.
Today, despite available treatments, more than 2 billion people are suffering from chronic infections with parasites, resulting in considerable morbidity [ 1 ]. Chronic infections develop because these parasites escape destruction by the immune system, arguably helped by their complex biology [ 2 ]. Parasitic infections and the host’s immune responses are the result of dynamic co-evolution of the host and the parasite’s complex life-cycle with each life stage resulting in a different interaction with the immune system [ 3 , 4 ]. Despite significant progress studying the biology of these parasites, there is still incomplete understanding of the interaction between the host’s immune response and these parasites [ 5 ].
Recent progress has been made in the development of transgenic parasites allowing for the structural and functional analysis of specific gene products [ 6 ], by for example, knocking-in or knocking-out target genes followed by functional analysis, in vivo [ 7 ]. For genetic manipulation of protozoan parasites ( Toxoplasma , Plasmodium and Leishmania ), robust systems were developed based on classical molecular biology methods such as microinjection, chemical transfection and electroporation, resulting in early advances in the understanding of the interactions between the immune system and these parasites. In contrast, for multicellular parasites these methods were not optimal and therefore, these parasites are now manipulated using transduction based on retroviral vectors.
Another breakthrough was the discovery that parasites possess the RNA-dependent gene silencing machinery and hence RNA interference (RNAi) has been applied to specifically downregulate genes, as opposed to producing a completely knocking-out of the gene of interest. For multicellular organisms such as Schistosoma mansoni lentivirus transduction was necessary to allow a highly efficient introduction of the foreign DNA [ 8 ]. Despite such progress, there are still limitations in gene manipulation approaches in multicellular parasites due to their diverse cellular elements and tissues, and complex life-cycles [ 9 ].
This review highlights the contributions made by studies on transgenic parasites to the understanding of host-parasite interactions and other applications of genetic manipulations in parasites were specifically omitted.
The databases PubMed and Web of Science were searched using keywords, including “transgenesis”, “parasite” and “immune response”. The search was conducted during the period of 2017 and 2018 and all the relevant scientific publications were screened following the procedure illustrated in the diagram below (Fig. 1 ). To meet the inclusion and exclusion criteria, only papers containing information on transgenic parasites and immune response study were included during the screen and non-published data or theses were not included for this study purpose.
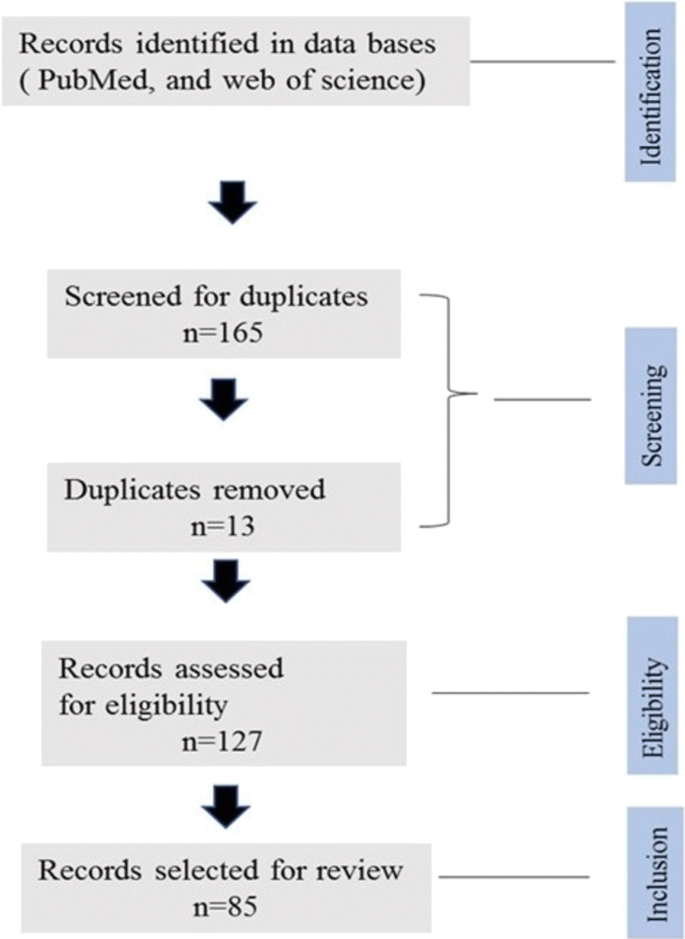
A flow diagram for the identification and screening of research articles for the current review
Expression of model antigens in parasites
One way of demonstrating protective cell-mediated immune responses is with the help of parasites transfected with putative protective antigens, followed by cell-transfer experiments to confirm the protective immune response. One special case of this approach consists of using antigens for which T cell receptor (TCR) transgenic mice have been generated [ 10 ]. These mice express the TCR specific for a peptide present in the model antigen presented in association with either MHC class II or I. For example, OT-II mouse—on a C57Bl/6 mouse background—express a TCR, which specifically recognises a peptide from chicken ovalbumin (OVA, amino acids 323–339) in association with MHC class II. In contrast, OT-I mouse express a transgene encoding a TCR specific for a short peptide fragment of OVA (amino acids 257–264) presented by MHC class I [ 11 ]. These mice can be used experimentally as a source of naïve T cells with a known specificity [ 12 ] and, hence, offer interesting tools to study T cell responses to these parasites. For example, transgenic Plasmodium berghei parasites were generated to express MHC class I- and II-restricted T cell peptide epitopes [ 13 ], recognised by TCR transgenic (HNT, DO11.10, and B6) mice, which were then used to elucidate the role of T cells in protective immunity to blood stage parasites. To achieve this, a T cell polytope was generated by artificially linking several T cell epitopes together including (i) MHC-I and II restricted epitopes from OVA, (ii) MHC-I restricted epitope from glycoprotein B of HSV-1, and (iii) MHC-I and II restricted epitopes from influenza virus strain PR8 hemagglutinin [ 13 ]. Blood stage of parasites transduced with this polytope were able to induce not only antigen-specific CD4 + T cells but, more unexpectedly, also antigen-specific CD8 + T cell responses via cross-presentation by CD8α + subsets of dendritic cells (DCs). While these antigen-specific CD8 + T cells did not contribute to protection against the blood stage of Plasmodium infection, they played a key role in the initiation of cerebral malaria (CM) and hence are important in the pathology of this infection [ 13 ]. This unexpected finding would have been very difficult to discover without the use of transgenic parasites, hereby demonstrating the usefulness of this technique for the analysis of immune responses to parasites.
Model antigens (i.e. antigens that are not naturally occurring in any pathogen), such as OVA, are frequently expressed in pathogens to investigate the major determinants and pathways influencing T cell responses. In contrast to pathogen-antigens, model antigens have never been subjected to evolutionary pressure and as a result they are unlikely to have been selected to induce biased immune responses. Thus, studying the immune response to these antigens not only allows for the use of many available reagents, but also facilitates the isolation of effects due to the antigen from these due to the pathogen. However, these model antigens are also artificial and therefore may not necessarily reflect the immune responses against pathogen antigens. Nevertheless, they represent useful tools in furthering our understanding of immune responses associated with parasites.
Other examples of the use of model antigens are studies showing that OT-II CD4 + and OT-I CD8 + T cells specifically recognize epitopes of OVA in Toxoplasma gondii including strains expressing OVA such as type I strain (expressing RH-OVA) and type II strains (expressing Pru-OVA). By injecting these transgenic parasites into mice, Pru-OVA can activate the DCs to produce cytokines within the draining lymph node and a large population of endogenous OVA-specific CD8 + T cells can be generated. Mice infected with RH-OVA have fewer DCs and OVA-specific CD8 + T cells [ 14 ]. The expression of model antigens in parasites allows not only the study of the major parasite determinants influencing immune responses, but also the exploration of the effects of the antigens’ subcellular localization on the induction of T cell response.
The expression of model antigens in different part of the parasite using transgenesis have been frequently used to determine the effect of antigen localisation on the host’s immune recognition. For example, P. berghei have been developed expressing recombinant proteins either in the cytosol or the parasitophorous vacuole membrane (PVM) to assess whether the sub-cellular location can influence T cell responses. OVA and mCherry conjugated with P. berghei heat-shock proteins such as HSP-70 were expressed in the cytoplasm. However, OVA gene sequence conjugated with the HEP17 (EXP1) promoter showed a low level of expression in the PVM [ 15 ]. Although both transgenic P. berghei lines induce OVA-specific CD8 + and CD4 + T cell responses, PVM-expressed OVA-HEP17 (EXP1) induced higher proliferation of OT-II and OT-I T cells than cytoplasma-expressed OVA-mCherry-HSP70, suggesting that antigen localisation within the parasite affects T cell recognition. Unconjugated OVA is not expressed in the cytoplasm of the parasite and more importantly here, the location and the degree of expression in the parasite also influence T cell responses [ 15 ]. The recognition of transgenic cytoplasmic OVA in Plasmodium by CD8 + T cells during different life-cycle stages of infection was also described [ 16 ]. However, those parasites were unable to induce a CD4 + T cell response in vivo [ 17 ]. A possible explanation for this dichotomy is that the localisation of the antigen in parasites drives the antigen processing pathways used in antigen presenting cells [ 18 ]. Furthermore, OVA expressed by blood stage P. berghei showed cross-presentation of the antigen by CD8α + antigen presenting cells in association with MHC class I and II inducing both CD8 + and CD4 + T cells [ 15 ].
Plasmodium spp. are not the only parasites for which the location of the antigen affects recognition by T cells. Indeed, Toxoplasma gondii has been engineered to express recombinant OVA either in the cytosol or to be secreted into the parasitophorous vacuole [ 19 ]. These studies demonstrated that only the secreted form of OVA, can stimulate CD4 + T cells and induce IFN-γ production (Fig. 2 ). Similarly, LacZ-expressing parasites containing CD8 T cell epitopes could only stimulate CD8 + T cell when the LacZ protein was expressed in a PV-secreted form but not when expressed as a cytosolic form [ 20 ]. In addition, T. gondii expressing secreted OVA in the PV, but not those that express a cytosolic OVA, were able to stimulate OVA-specific CD4 + T cells [ 21 ]. Therefore, antigens secreted in the PV are more effective at activating both CD4 and CD8 T cell. PV-secreted antigens might be more efficiently released from infected cells and hence more antigen could be available to be processed through the MHC II pathway. Similarly, PV-secreted antigens are transported more effectively from the PV into the host cell cytoplasm possibly through cross-presentation dependent of the TAP transporter.
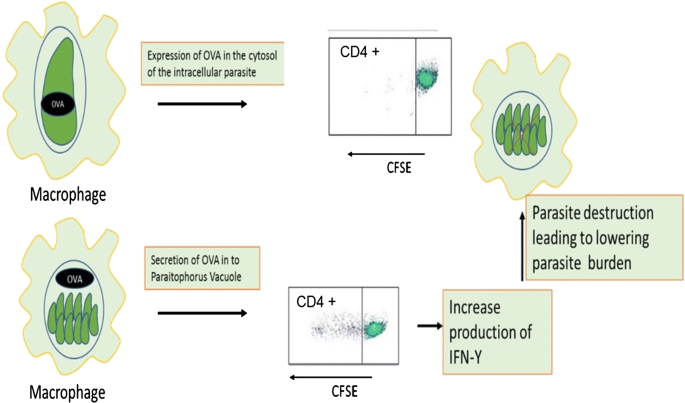
The site of OVA expression inside the transgenic parasite has influenced the cellular immune responses in which cytosolic OVA leads to stimulation of CD4 + T cells and IFN-γ production. To confirm whether OVA expressing transgenic T. gondii can induce T cell proliferation, CD4 + T cells were labelled with CFSE and the in vitro response of the cells showed the cytosolic OVA failed to show any T cell response, but OVA expressed at the parasitophorous vacuole induced T cell proliferation [ 25 ]
Furthermore, Leishmania spp. have also been used to study the effect of expressed antigens at different locations on the induction of T cell responses. For example, OVA has been used as a reporter gene by integrating it into the genomic DNA of Leishmania donovani , allowing the isolation of OVA-expressing amastigotes. Mice adoptively transferred with OVA-specific OT-I T cells and infected with OVA-transgenic parasite demonstrated that OVA could be recognised in the context of MHC class I and in the same study a reduction in hepatic and splenic parasite burden was observed [ 22 ]. Furthermore, in a follow-up experiment, OVA expressed on the plasma membrane led to a significant activation of CD4 + T cells [ 23 ]. The ability of a fusion protein containing OVA (NT-OVA), expressed by transgenic parasites in either a secreted form or an intracellular form, to stimulate OT-I CD8 + T cells was investigated [ 24 ]. Dendritic cells infected by Leishmania major transfected with secreted NT-OVA could activate naïve OT-I cells while non-secreted (i.e. intracellular) NT-OVA was unable to achieve this outcome, possibly because less OVA was available in the phagosomes [ 24 ]. Indeed, high concentrations of NT-OVA might be required in the phagosomes, to allow for the relatively inefficient cross-presentation of OVA present in the phagosome (where the parasite resides), through the MHC I pathway. DCs pulsed with heat-killed parasites were also unable to activate naïve OT-I cells, possibly because not enough antigen can accumulate in the phagosomes and hence transit via cross-presentation to MHC I. Interestingly, infected macrophages, which generally lack the antigen cross-presentation pathway, were unable to activate naïve OT-I cells when infected with L. major transfected with either the secreted or the intracellular form of NT-OVA (Fig. 3 ), corroborating the results suggesting the use of cross-presentation in this process. Macrophages were able to activate primed OT-I cells but 20 times less efficiently, compared to DCs. Taken together, these results suggest that L. major antigens secreted in the phagosomes of DCs, but not macrophages, can cross-present antigenic peptides to MHC I leading to CD8 + T cells activation, while the local accumulation of intracellular antigens is insufficient to allow cross-presentation and hence no CD8 activation can occur. Interestingly, as noted above, similar conclusions were made for T. gondii except that in contrast to the Leishmania , T. gondii antigens in the PV do not seem to go directly into the MHC II antigen processing pathway.
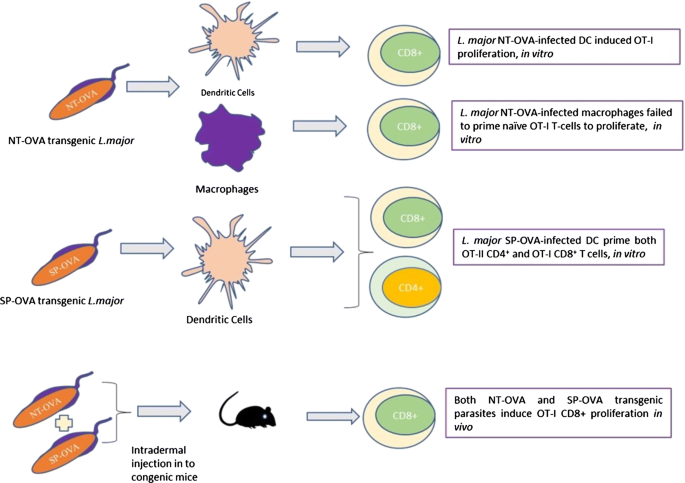
The expression of two OVA epitopes (NT-OVA and SP-OVA) in L. major parasite showed different type of T cell responses when exposed to dendritic cells and macrophages in vitro separately. Whereas, injection of both OVA epitope transgenic parasites into mice after adoptive transfer of OVA specific OT-I T cells showed only an induction of CD8 + T cells in vivo
Expression of reporter genes for parasite tracking and immune cell characterization
The advent of model reporter gene technology has facilitated the understanding of many cellular responses to parasites with distinct phenotypic properties from the system being investigated.
Due to various limitations of light microscopy-based in vivo imaging techniques, the direct investigation of different events in the host has been impossible until recently [ 25 ]. One of the earliest studies of immune responses in vivo involved adoptive transfer of T cells, B cells, and DCs to a recipient host [ 26 ]. In the same context, different fluorescent dyes such as CFSE have been used for the labelling and short-term tracking of the cells as they migrate in the host. However, as cell division or activation results in dilution of the dye, tracking of the cell population over longer time periods was not possible in this model [ 25 ]. To overcome such difficulties, different fluorescent proteins have been expressed under promoters specific for various cell types such as, CD4 + T cells, CD8 + T cells, macrophages, DCs, and neutrophils and this has allowed long-term investigation of cell trafficking during in vivo immune responses [ 25 ]. This approach has been combined with the expression of different reporter genes in the parasite [ 27 ]. For example, transgenic T. gondii expressing luciferase enabled real-time monitoring of an infection in vivo [ 6 ]. In addition, several reporter molecules have been generated for cytokines such as IFN-γ, IL-2, IL-10 and IL-12 to understand how their production can be visualized and their association with host cells in vivo ascertained [ 28 ]. There has also been recent progress in using real-time imaging of host-pathogen interactions, for example such approach has been reported in Toxoplasma , Plasmodium and Leishmania [ 29 ].
Toxoplasma gondii expressing fluorescent markers have been used to distinguish infected macrophages, uninfected macrophages and DCs. Such transgenic parasites are also used to characterise the immunological phenotypes of these cells under flow cytometry [ 30 , 31 ].
Another application for fluorescent marker-expressing parasites is the unravelling of the mechanisms leading to CM. For example, blood parasitemia and sequestration of parasites in the brain vasculature is the main predisposing factor for CM and both CD8 + and CD4 + T cells play important roles not only in the control of parasitemia, but also in the development of CM [ 32 ]. CD8 + T cells sequester in the brain tissue following P. berghei infection causing damage to the endothelial tissue of the brain through the production of perforins [ 33 ]. The association between Treg and CD8 + T cell recruitment in the pathogenesis of experimental CM was studied using P. berghei expressing luciferase and green fluorescent protein. Depletion of Treg cells with anti-CD25 monoclonal antibody leads to protection of mice from experimental cerebral malaria. In addition, the accumulation of parasites in the brain vasculature was reduced in these infected mice, resulting in a significant reduction of parasite burden. Mice lacking Treg cells showed higher numbers of activated CD4 + and CD8 + T cells in spleen and lymph nodes. However, CD8 + T cell recruitment to the brain was selectively reduced in these mice [ 34 ]. Thus, transgenic parasites have not only helped unravel the host’s immune response to wards the parasite but also the immune mechanisms contributing to a major aspect of the pathology of malaria.
Furthermore, a luciferase transgenic L. donovani has also been used to demonstrate a role for the Ras-related protein, Rab5, in the regulation of the phagosome-endosome fusion and how these subcellular compartments kill intracellular parasites [ 35 ]. Through expression of GFP in L. major , the temporal difference of the parasite antigens on the draining lymph node is demonstrated [ 36 ] and similar studies showed the role of lipophosphoglycan (LPG) in inducing DC-mediated pro-inflammatory responses using Leishmania mexicana LPG1 −/− mutants and this study reported high level of pro-inflammatory cytokine gene expression in the absence of LPG [ 37 ]. Therefore, the expression of reporter proteins by parasites has been important to track the events of parasite dissemination and replication during infection and such transgenesis technology has greatly increased the accessibility of improved humanized mouse models to investigate the protection efficacy of different parasite origin antigens, as discussed in the next section.
Expression of antigens from other parasite species
In order to show that particular antigens are important targets for antibody-mediated protection, transgenic parasites have become a very useful tool (Fig. 4 ). For example antibodies play a significant role in the inhibition of blood stage merozoites by targeting the major protein component of MSP-1 19 and therefore, by replacing the P. berghei allele with the P. falciparum one, MSP-1 19 antibodies with a high level of inhibitory potential against the human parasite, were generated in mice. As a result, during challenge trials, a positive correlation between protection of mice from the blood stage malaria and the level of the surface protein expressed was observed. Moreover, full-length protein variants have been expressed and characterized in a transgenic P. berghei lines and patent infection of mice by these transgenic lines showed production of protective antibodies [ 38 ].
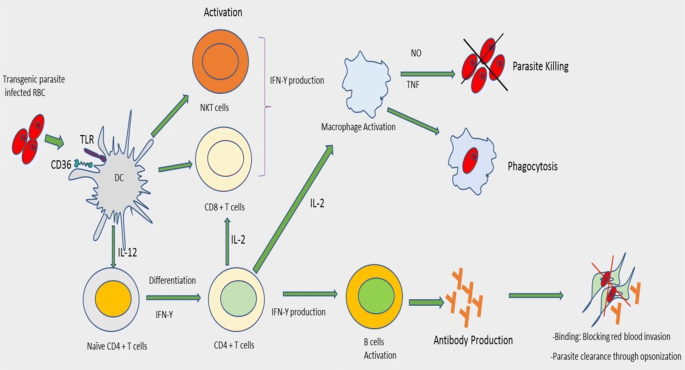
Transgenic P. berghei parasite expressing a circumsporozoite protein induces a strong antibody production and protection efficiency. Briefly, the transgenic malaria parasite lines infect the RBC of C57BL/6 mice and leads to the activation of B cells and IFN-γ production by CD4 + T cells. Passive transfer of antibodies to naïve recipient mice confers protection through opsonization process
Plasmodium berghei , P. chabaudi and P. yoelii are three malaria species that infect mice and are most commonly used due to their shared pathological features with P. falciparum , the most pathogenic strains of human malaria parasite [ 39 ]. In order to investigate the potential of the circumsporozoite protein (CSP) as a vaccine antigen in vivo , the csp gene of rodent malaria parasites was replaced with the csp genes from human parasites such as P. falciparum and P. vivax . The chimeric rodent parasites could produce sporozoites in Anopheles stephensi mosquitoes, capable of infecting human and rodent hepatocytes [ 40 ]. Thus, by expressing recombinant antigens from a human parasite into a mouse parasite, it is possible to take advantage of a mouse model to identify neutralising immune responses in a mouse model and hence discover potential vaccine candidates for human malaria.
Expression of transgenes by parasites to improve their immunogenicity
Many parasites use immunomodulatory mechanisms to ensure their survival in the host by crippling the host’s immune response, most often through the production of immunomodulatory molecules [ 41 ], which bind or interact with host immune cells [ 42 ]. Others have developed ways of remaining silent by virtue of low or absent expression of pathogen associated molecular patterns (PAMPs). Thus, when parasites evade the immune system by suppressing expression of PAMPs, it might be possible to express PAMPs transgenes from other organisms, and hence attract the attention of the immune system to the parasite, making them more immunogenic. To unravel this issue, transgenic T. cruzi expressing an exogenous PAMP with the ability to activate the innate immune system such as (i) Salmonella typhimurium flagellin (FliC), (ii) Neisseria meningitidis (FAM18 strain) porin (PorB) or (iii) T. cruzi paraflagellar rod protein 4 (PAR4) genes have been generated. Not surprisingly, strong innate immune responses were observed when mice were infected with these transgenic parasites and the PAMPs activated innate immune cells and these activated immune cells played an important role in controlling T. cruzi infection [ 43 ]. These results suggest that a relative deficiency of PAMPs in T. cruzi helps the pathogen to survive in the host. These studies also showed that inoculation of exogenous PAMPs with T. cruzi and under continuous expression can induce strong CD8 + T cell responses which ultimately leads to the control and clearance of infection (Fig. 5 ). A similar study aimed at optimal immune control and clearance of parasites persisted in the host also showed that transgenic T. cruzi expressing flagellar protein PAR4 was more effective at inducing PAR4-specific CD8 + T cell responses, which improved protection during subsequent parasite challenges [ 43 ]. Hence, these experiments demonstrate that the relative absence of PAMPs in T. cruzi can be harnessed to study the importance of PAMPs from other sources, in the induction of adaptive immunity.
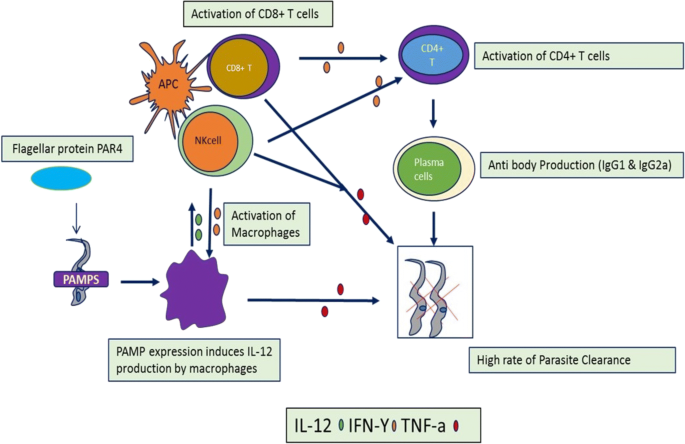
Expression of flagellar protein PAR4 in T. cruzi causes the activation of macrophages, CD8 + T cells and NKT cells which leads to the high rate of parasite destruction from the circulation through the production of different cytokines having direct effect such as TNF-a and through the activation of plasma cells and production of protective antibodies
The two functionally distinct T helper cell populations, which determine the Leishmania infections are the Th1 cells known for secretion of IL-2 and IFN-γ cytokines and Th2 cells known for the secretions of IL-4, IL-10 and IL-13. The susceptibility to Leishmania infection is associated with Th2 proliferation and secretion of their cytokine signatures mentioned above and resistance to infection is maintained by the secretion IFN-γ by CD4 + Th1 helper cells [ 44 ]. However, there is no clear evidence how the parasite skewed the immune response and leads into protection and disease progression. L. major secreting the mouse cytokine granulocyte-macrophage colony-stimulating factor (GM-CSF) is engineered to induce protective host immune responses [ 45 ]. Indeed, GM-CSF induces secretion of many pro-inflammatory cytokines such as IL-1β, IL-18 and IL-6 by macrophages and, as a result, transgenic parasites expressing GM-CSF failed to survive. Transgenic Leishmania spp. engineered to produce monocyte chemoattractant protein 1 (MCP-1) were less pathogenic in susceptible BALB/c mice in vivo , with significant reduction in lesion size compared to wild-type parasites [ 46 ]. In addition, parasites producing MCP-1 caused CCR2 positive macrophage recruitment, but the innate immune response was less efficient at inducing protective immunity against subsequent challenges.
In another application, transgenic parasites can also be used as vaccine vehicles. Indeed, one study showed the development of a novel system for the expression of nematode secreted proteins in another parasite such as Trypanosoma musculi, a natural parasite of mice. Furthermore, acetylcholinesterase from Nippostrongylus brasiliensis was engineered in trypanosomes and these transgenic trypanosomes were able to induce a protective immune response against N. brasiliensis , by altering the cytokine environment. In the same study, immune cells exposed to acetylcholinesterase expressing parasites in vivo showed activation of macrophages, production of high levels of nitric oxide and decreasing arginase activity [ 47 ].
In summary, the transgenesis of proteins that improve immunogenicity of the parasite have given us insights not only in how parasites evade the immune system, for example by reducing the amount of danger signals they express but more importantly also in the fundamental mechanisms of immune regulation, particularly the role of key cytokines. Some of these engineered parasites might even be used as vaccine vehicles, although this would require passing considerable regulatory hurdles.
Deleting and knocking-down parasite genes to evaluate their effect on immune responses
Deletion mutants of parasites have been used to investigate the role of critical molecules in the binding of the parasite to the host cells and the effect of antibodies to these molecules in inhibiting this process. Indeed, studies in human malaria showed that erythrocyte binding-like (EBL) proteins and reticulocyte binding-like (RBL or PfRh) proteins are involved in erythrocyte bindings and are targets of human invasion inhibitory antibodies in addition to being important components of acquired protective immunity [ 48 ]. A construct of P. falciparum lines in which eba-175 , eba-181 , and eba-140 genes were knocked out by targeted disruption, showed that the EBL and PfRh proteins functionally interact during merozoite invasion. Further evidence showed that PfRh and EBL proteins induced antibodies that potently blocked merozoite invasions and hence these antigens could potentially be used as vaccine candidates [ 48 , 49 ]. The targeted disruption of key genes to establish a role for antibodies to specific proteins can only be used when knock-out parasites are viable, as is the case with the eba genes. However, in some instances such as the MSP-1 protein a knock-out strategy can be unsuccessful [ 50 ], for example if the protein is essential for parasite survival. In such cases researchers have resorted to the more complex approach of expressing the protein from one species of malaria into another (see below), to demonstrate the role of antibodies in protection. Another alternative is the use of RNAi, which by its nature downregulates the expression of the protein but does not abolish expression altogether.
The ability of parasites to modulate immune responses is often mediated by the production of immunomodulatory molecules. Hence, there is a growing interest in the characterization of the mediators released by the parasites and analysing how such molecules can redirect the host’s immune response [ 42 ]. This might also have a tremendous advantage in developing unique and targeted therapies not only against the parasites [ 51 ], but also potentially for the treatment of other immune-mediated conditions.
Parasites, such as Schistosoma mansoni , are among the pathogenic organisms that invade diverse organ systems such as the lymphatic system, the gastro-intestinal tract and the vascular system with multiple immunomodulatory lines of attack on the immune system. Co-evolution of parasites and their hosts’ immune system has resulted in a delicate balance that allows chronic infections to be maintained without provoking fatal immunopathology or overwhelming infection in the host [ 52 , 53 ]. S. mansoni egg proteins including Omega-1, IPSE, and kappa-5 are the main contributors of egg-induced immune regulation [ 54 ]. The effect of soluble egg antigens (SEA), such as Omega-1 , is attributed to an altered interaction of DCs with Th cells and the initiation of mRNA and rRNA degradation. This leads to abolished protein-expression by DCs. These interactions and the immunomodulatory mechanisms can be investigated by downregulating Omega-1 in S. mansoni eggs and studying the effect in vivo [ 8 ]. Omega-1 alone can induce a Th2 response in vitro and in vivo through the conditioning of DCs. Indeed, the depletion of this protein totally abrogates the maturation of T cells induced by schistosome SEA in vitro [ 55 ]. As mentioned in the introduction, multicellular parasites are difficult to transfect using classical molecular techniques and of the require lentivirus-mediated transgenesis. Lentiviral transduction system mediated knocking-down of Omega-1 in S. mansoni eggs leads to the production of IFN-γ [ 8 ] (Fig. 6 ). In the same study, lung cell suspensions from naïve mice, which were intravenously injected with wild-type and empty vector transduced S. mansoni eggs revealed a characteristic Th2 cytokine profile (IL-4, IL-5, IL-6, IL-10 & IL-13) with low levels of Th1 (IL-1α, IL-12, INF-γ, TNF-α), and Th17 cytokines (IL-17, IL-22). Moreover, intravenous injection of wild-type S. mansoni eggs increased numbers of eosinophils, granulocytes, T helper cells, alveolar APCs and B cells [ 8 ]. In contrast, mice injected with Omega-1 knock-down eggs had a cytokine profile characterised by a slight increase in Th1 cytokines, such as TNF-α. However, no significant difference in the levels of Th1, Th2, and Th17 cytokines was observed between the Omega-1 knock-down eggs and wild-type or the empty vector controls. However, Omega-1 knock-down parasites had reduced pathology in the lungs, suggesting an effect on granuloma formation. Although Omega-1 is the principal component of SEA, which conditions DCs for priming Th2 responses, it is not the only glycoprotein in SEA that can induce these responses. Other egg proteins, such as IPSE and Kappa-5 also play a role in immunomodulation of the host’s immune response. Hence, knock-down of Ipse and Kappa-5 leads to a slight decrease in IL-2 and IL-10 production and an increase in secretion of IL-1 and IL-6 but, the overall difference in the level of secreted cytokines was not significant for either of the SEA knock-down groups.
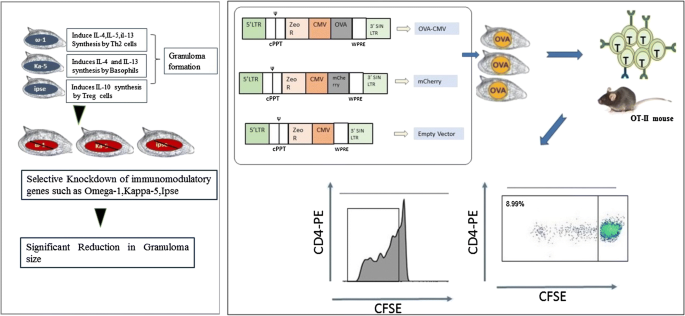
Schistosoma mansoni eggs transduced with lentiviruses containing shRNAmir showed a significant reduction the size of granuloma comparing with the untraduced eggs [ 8 ] and the expression of chicken ovalbumin in S. mansoni eggs after delivery of the OVA transgene through the lentiviral transduction system leads in to the recognition of the OVA by OT-II T cells in vitro
In recent years the CRISPR/Cas9-based genome edition tools have become a standard way of disrupting key genes in order to study their effect. However, most of the studies so far have used this technique to investigate the parasite biology rather than host-parasite interactions, which fall outside of the scope of this study. While one can expect many future studies to include CRISPR/Cas9-based genome edition tools in the study of immune responses to parasite, the only study so far targeted Omega-1 of S. mansoni eggs and the results conformed these discovered by downregulating this gene using the RNAi/lentiviral transduction system, namely a reduction of pulmonary granuloma [ 56 ].
In another parasite example, deletion of L. mexicana cysteine peptidase B in these parasites resulted in similar lesion-development compared to wild-type parasites in the early stages of infections [ 57 ]. However, mice infected with CPB -/- parasites cleared the infection after inducing a STAT4 and IL-12-dependent Th1 response, indicating that cysteine peptidase B suppresses the immune response [ 58 ].
Finally, disruption of the conserved 6-Cys B gene family, which plays a role during development of sexual stages of Babesia bovis, resulted in inhibiting parasite invasion of red blood cells [ 59 ]. Such novel gene manipulation approach could generate attenuated parasites usable as vaccines against babesiosis.
Taken together these experiments demonstrate the usefulness of different gene-disruption and downregulation technologies for the study of gene function of parasites including these highlighted here in relation to host-parasite interactions. While gene disruption offers a more absolute effect because the protein of interest is completely absent, gene downregulation has the advantage that if the gene is essential the parasite might survive although in an impaired state and hence an effect might still be observable in that case.
Summary and future directions
As outlined in this review, recent advances in transgenesis of complex parasites have allowed significant progress towards understanding the immunobiology of a range of parasites. These advances could potentially also be applied to even larger parasites, such as parasitic nematodes. Indeed, the tracking of nematode-specific T cell responses has so far been a challenge [ 60 ]. The use of nematodes expressing model antigens, such as OVA, might help in this respect, particularly if combined with T cell receptor transgenic mice. Studies over the last two decades involving in vivo depletion of specific cells, cytokines and their receptors showed that the immune response to nematodes in a murine model is mainly dependent CD4 + T cells [ 61 , 62 ]. Parasitic nematodes induce immunomodulatory mechanisms to invade their host and sustain their infectivity [ 63 ]. While there is a general mechanism of immunomodulation by nematodes which involves the release of molecules capable of manipulating host immune responses, the nature, and mechanisms of altering the host’s immunity is different from one parasitic nematode to the other [ 41 ]. Therefore, to help with this endeavour knock-down or deletion of specific genes suspected to have effects on the immune system can be generated and used to confirm their respective roles.
Importantly, parasites in which one key immune mechanism has been eliminated could potentially reveal additional mechanisms that are normally masked by the overpowering effect of the main immune mechanism. The use of transgenic parasites in studying immune response is summarised in Table 1 . Therefore, we forwarded the following learning points.
We have reviewed current advances focusing on the use of transgenesis as a tool in the study of host-parasite interactions.
The transgenesis approach is now becoming a novel technique in the integration of foreign genes reliably into the host genome for functional analysis of the immune response in vivo .
To date, few transgenic parasites have been developed and improvements in the advancement of this field is required to increase our knowledge of parasite immunology.
We foresee that such advancement in transgenesis technology could pave the way to rapid developments in functional genomics as it relates to host-parasite interactions. For example, high throughput insertional mutagenesis, expansion of RNAi for in vivo studies might soon become a practical reality.
We also expect the advances achieved thus far can be adapted in a wide range of pathogenic parasites to understand their nature of interaction with the host.
Conclusions
In conclusion, transgenic parasites have been utilised in a wide range of applications to investigate complex host-parasite interactions in vivo . Indeed, using this versatile technology, it is now possible to unravel the detailed immune response to parasites. While this review highlights how transgenic parasites have influenced our understanding of the host-parasite interaction, many additional questions remain and establishing these advanced transgenesis methods for applications in an ever-increasing variety of parasite species will undoubtedly result in the discovery of fascinating insights about the interactions of parasites with the immune system of their hosts.
Availability of data and materials
Not applicable.
Abbreviations
RNA interference
T cell receptor
major histo compartment I
major histo compartment II
dendritic cells
cerebral malaria
parasitophorous vacuole membrane
lipophosphoglycan
chicken ovalbumin
circumsporozoite protein
merozoite surface protein
pathogen-associated molecular pattern
soluble egg antigens
messenger RNA
ribosomal RNA
granulocyte-macrophage colony-stimulating factor
Maizels RM, Yazdanbakhsh M. Immune regulation by helminth parasites: cellular and molecular mechanism. Nat Rev Immunol. 2003;3:733–44.
Article CAS PubMed Google Scholar
Pearce BEJ, Sher A. Mechanisms of immune evasion in schistosomiasis. Contrib Microbiol Immunol. 1987;8:219–32.
CAS PubMed Google Scholar
Anthony RM, Rutitzky I, Urban JF, Stadecker MJ, Gause WC. Protective immune mechanisms in helminth infection. Nat Rev Immunol. 2007;7:975–87.
Article CAS PubMed PubMed Central Google Scholar
Andrew SM, Maria IA, Edward JP. Immunology of parasitic helminth infections. Infect Immun. 2002;70:427–33.
Article CAS Google Scholar
Brindley PJ, Mitreva M, Ghedin E, Lustigman S. Helminth genomics: the implications for human health. PLoS Negl Trop Dis. 2009;3:e538.
Article PubMed PubMed Central Google Scholar
Saeij JPJ, Boyle JP, Grigg ME, Arrizabalaga G, Boothroyd JC. Bioluminescence imaging of Toxoplasma gondii infection in living mice reveals dramatic differences between strain. Infect Immun. 2005;73:695–702.
Reinke AW, Troemel ER. The development of genetic modification techniques in intracellular parasites and potential applications to microsporidia. PLoS Pathog. 2015;11:e1005283.
Article PubMed PubMed Central CAS Google Scholar
Hagen J, Young ND, Every AL, Pagel CN, Schnoeller C, Scheerlinck JY, Gasser RB, et al. Omega-1 knockdown in Schistosoma mansoni eggs by lentivirus transduction reduces granuloma size in vivo . Nat Commun. 2014;5:1–9.
Article Google Scholar
Boyle JP, Yoshino TP. Gene manipulation in parasitic helminths: review. Int J Parasitol. 2003;33:1259–68.
Kouskoff V, Signorelli K, Benoist C, Mathis D. Cassette vectors directing expression of T cell receptor genes in transgenic mice. Immunol Methods. 1995;180:273–80.
Clarke SR, Barnden M, Kurts C, Carbone FR, Miller JF, Heath WR. Characterization of the ovalbumin-specific TCR transgenic line OT-I:MHC elements for positive and negative selection. Immunol cell Biol. 2000;78:110–7.
Wright KO, Murray DA, Crispe NI, Pierce RH. Quantitative PCR for detection of the OT-1 transgene. BMC Immunol. 2005;6:20.
Lundie RJ, de Koning-Ward TF, Davey GM, Nie CQ, Hansen DS, Lau LS, et al. Blood-stage plasmodium infection induces CD8 + T lymphocytes to parasite-expressed antigens, largely regulated by CD8α + dendritic cells. Proc Natl Acad Sci USA. 2008;105:14509–14.
Tait ED, Jordan KA, Dupont CD, Harris TH, Gregg B, Wilson EH, et al. Virulence of Toxoplasma gondii is associated with distinct dendritic cell responses and reduced numbers of activated CD8 + T cells. J Immunol. 2010;185:1502–12.
Lin JW, Shaw TN, Annoura T, Fougère A, Bouchier P, Chevalley-Maurel S, et al. The subcellular location of ovalbumin in Plasmodium berghei blood stages influences the magnitude of T-cell responses. Infect Immun. 2014;82:4654–65.
Kimura K, Kimura D, Matsushima Y, Miyakoda M, Honma K, Yuda M. CD8 + T cells specific for a malaria cytoplasmic antigen form clusters around infected hepatocytes and are protective at the liver stage of infection. Infect Immun. 2003;8:3825–34.
Google Scholar
Lundie RJ, Young J, Davey GM, Villadangos JA, Carbone FR, Heath WR. Blood-stage Plasmodium berghei infection leads to short-lived parasite-associated antigen presentation by dendritic cells. Eur J Immunol. 2010;40:1674–81.
Von der Weid T, Kopf M, Kochler G, Langhorne J. The immune response to Plasmodium chabaudi malaria in interleukin-4-deficient mice. Eur J Immunol. 1994;24:2285–93.
Article PubMed Google Scholar
Dzierszinski FS, Hunter CA. Advances in the use of genetically engineered parasites to study immunity to Toxoplasma gondii . Parasite Immunol. 2008;30:235–44.
Seeber F, Boothroyd JC. Escherichia coli beta-galactosidase as an in vitro and in vivo reporter enzyme and stable transfection marker in the intracellular protozoan parasite Toxoplasma gondii . Gene. 1996;169:39–45.
Pepper M, Dzierszinski F, Crawford A, Hunter CA, Roos D. Development of a system to study CD4 + T cell responses to transgenic ovalbumin-expressing Toxoplasma gondii during toxoplasmosis. Infect Immun. 2004;72:7240–6.
Polley R, Stager S, Prickett S, Maroof A, Zubairi S, Smith DF, et al. Adoptive immunotherapy against experimental visceral leishmaniasis with CD8 + T cells requires the presence of cognate antigen. Infect Immun. 2006;74:773–6.
Prickett S, Gray PM, Colpitts SL, Scott P, Kaye PM, Smith DF. In vivo recognition of ovalbumin expressed by transgenic Leishmania is determined by its subcellular localization. J Immunol. 2006;176:4826–33.
Bertholet S, Debrabant A, Afrin F, Caler E, Mendez S, Tabbara KS, et al. Antigen requirements for efficient priming of CD8 + T cells by Leishmania major -infected dendritic cells. Infect Immun. 2005;73:6620–8.
John B, Weninger W, Hunter CA. Advances in imaging the innate and adaptive immune response to Toxoplasma gondii . Future Microbiol. 2010;5:1321–8.
Bousso P, Robey E. Dynamics of CD8 + T cell priming by dendritic cells in intact lymph nodes. Nat Immunol. 2003;4:579–85.
Gubbels MJ, Striepen B. Studying the cell biology of apicomplexan parasites using fluorescent proteins. Microsc Microanal. 2004;10:568–79.
Reinhardt RL, Hong S, Kang SJ, Wang ZE, Locksley RM. Visualization of IL-12/23p40 in vivo reveals immunostimulatory dendritic cell migrants that promote Th1 differentiation. J Immunol. 2006;177:1618–27.
Dube A, Gupta R, Singh N. Reporter genes facilitating discovery of drugs targeting protozoan parasites. Trends Parasitol. 2009;25:432–9.
Wille U, Villegas EN, Striepen B, Roos DS, Hunter CA. Interleukin-10 does not contribute to the pathogenesis of a virulent strain of Toxoplasma gondii . Parasite Immunol. 2001;23:291–6.
Gubbels MJ, Striepen B, Shastri N, Turkoz M, Robey EA. Class I major histocompatibility complex presentation of antigens that escape from the parasitophorous vacuole of Toxoplasma gondii . Infect Immun. 2005;73:703–11.
Weidanz WP, Melancon-Kaplan J, Cavacini LA. Cell-mediated immunity to the asexual blood stages of malarial parasites: animal models. Immunol Lett. 1990;25:87–95.
Belnoue E, Kayibanda M, Vigario AM, Deschemin JC, Van Rooijen N, Viguier M. On the pathogenic role of brain-sequestered αβ CD8 + T cells in experimental cerebral malaria. J Immunol. 2002;69:6369–75.
Amante FH, Stanley AC, Randall LM, Zhou Y, Haque A, McSweeney K, et al. A role for natural regulatory T cells in the pathogenesis of experimental cerebral malaria. Am J Pathol. 2007;171:548–59.
Duclos S, Diez R, Garin J, Papadopoulou B, Descoteaux A, Stenmark H, et al. Rab5 regulates the kiss and run fusion between phagosomes and endosomes and the acquisition of phagosome leishmanicidal properties in RAW 264.7 macrophages. J Cell Sci. 2003;113:3531–41.
Misslitz AC, Kerstin B, Dorothee H, Christoph L, Thomas K, Toni A. Two waves of antigen-containing dendritic cells in vivo in experimental Leishmania major infection. Eur J Immunol. 2004;34:715–25.
Aebischer T, Bennett CL, Pelizzola M, Vizzardelli C, Pavelka N, Urbano M, et al. A critical role for lipophosphoglycan in proinflammatory responses of dendritic cells to Leishmania mexicana . Eur J Immunol. 2005;35:476–86.
De Koning-Ward TF, O’Donnell RA, Drew DR, Thomson R, Speed TP, Crabb BS, et al. A new rodent model to assess blood stage immunity to the Plasmodium falciparum antigen merozoite surface protein 119 reveals a protective role for invasion inhibitory antibodies. J Exp Med. 2003;198:869–75.
Thompson J, Millington OR, Garside P, Brewer JM. What can transgenic parasites tell us about the development of Plasmodium -specific immune responses? Par Immunol. 2008;30:223–33.
Mogollon CM, Van Pul FJA, Miyazaki S, Imai T, Ramesar J, Salman AM, et al. Chimeric Plasmodium falciparum parasites expressing Plasmodium vivax circumsporozoite protein fail to produce salivary gland sporozoites. Malar J. 2018;17:288.
Cooper D, Eleftherianos I. Parasitic nematode immunomodulatory strategies: recent advances and perspectives. J Pathog. 2016;5:58.
Lightowlers MW, Rickard MD. Excretory-secretory products of helminth parasites: effects on host immune responses. Parasitology. 1988;96:123–66.
Kurup SP, Tarleton RL, Diseases EG. Perpetual expression of PAMPs necessary for optimal immune control and clearance of a persistent pathogen. Nat Commun. 2014;4:2616.
Nylen S, Gautam S. Immunological perspectives of leishmaniasis. J Glob Infect Dis. 2010;2:135–46.
Dumas C, Muyombwe RAG, Roy G, Matte C, Ouellette M, Olivier M, et al. Recombinant Leishmania major secreting biologically active granulocyte-macrophage colony-stimulating factor survives poorly in macrophages in vitro and delays disease development in mice. Infect Immun. 2003;71:6499–509.
Conrad SM, Strauss-Ayali D, Field AE, Mack M, Mosser DM. Leishmania - derived murine monocyte chemoattractant protein 1 enhances the recruitment of a restrictive population of CC chemokine receptor 2-positive macrophages. Infect Immun. 2007;75:653–65.
Vaux R, Corinna S, Rita B, Luke BR, Jana H, Kleoniki G, et al. Modulation of the immune response by nematode secreted acetylcholinesterase revealed by heterologous expression in Trypanosoma musculi . PLoS Pathog. 2016;12:e1005998.
Lopaticki S, Maier AG, Thompson J, Wilson DW, Tham W, Triglia T, et al. Reticulocyte and erythrocyte binding-like proteins function cooperatively in invasion of human erythrocytes by malaria parasites. Infect Immun. 2011;79:1107–17.
Gicheru N, Reiling L, Jack S, Wilson DW, Lopaticki S, Alan F, et al. Erythrocyte-binding antigens of Plasmodium falciparum are targets of human inhibitory antibodies and function to evade naturally acquired immunity. J Immunol. 2013;191:785–94.
Cowman AF, Crabb BS. Invasion of red blood cells by malaria parasites: review. Cell. 2006;124:755–66.
Jackson JA, Turner JD, Kamal M, Wright V, Bickle Q, Else KJ, et al. Gastrointestinal nematode infection is associated with variation in innate immune responsiveness. Microbes Infect. 2006;8:487–92.
Maizels RM, Taylor MD, Judith E. Helminth parasites-masters of regulation. Immunol Rev. 2004;4:89–117.
Zaccone P, Burton O, Miller N, Jones FM, Dunne DW, Cooke A. Schistosoma mansoni egg antigens induce Treg that participate in diabetes prevention in NOD mice. Eur J Immunol. 2009;39:1098–107.
Hams E, Aviello G, Fallon PG. The schistosoma granuloma: friend or foe? Front Immunol. 2013;4:89.
Everts B, Perona-Wright G, Smits HH, Hokke CH, Van der Ham AJ, Fitzsimmons CM, et al. Omega-1, a glycoprotein secreted by Schistosoma mansoni eggs, drives Th2 responses. J Exp Med. 2009;206:1673–80.
Wannaporn I, Victoria HM, Shannon EK, Avril C, Gabriel R, Geetha S, et al. Programmed genome editing of the omega-1 ribonuclease of the blood fluke, Schistosoma mansoni . eLife. 2019;8:e41337.
Cameron P, McGachy A, Anderson M, Paul A, Coombs GH, Mottram JC, et al. Inhibition of lipopolysaccharide-induced macrophage IL-12 production by Leishmania mexicana amastigotes: the role of cysteine peptidases and the NF-κB signalling pathway. J Immunol. 2004;173:3297–304.
Beattie L, Evans M, Kaye PM, Smith DF. Transgenic leishmania and the immune response to infection. Parasite Immunol. 2008;30:4.
Heba FA, Brian MC, Carlos ES. Transgenic babesia bovis lacking 6-cys sexual-stage genes as the foundation for non-transmissible live vaccines against bovine babesiosis. Ticks Tick-borne Dis. 2019;10:722–8.
Pearce EJ. An increased understanding of T-cell responses during helminth infections. Parasite Immunol. 2006;28:235–69.
Szabo SJ, Sullivan BM, Peng SL, Glimcher LH. Molecular mechanisms regulating Th1 immune responses. Annu Rev Immunol. 2004;21:713–58.
Mowen KA, Glimcher LH. Signalling pathways in Th2 development. Immunol Rev. 2004;202:203–22.
Hartmann S, Lucius R. Modulation of host immune responses by nematode cystatins. Int J Parasitol. 2003;33:1291–302.
Jiang B, Qin L, Du Y, Peng N, Chen L, Chen Z, Chen X. Transgenic Plasmodium that expresses HIV-1 Gag elicits immunity and protects mice against vaccinia virus-gag and malarial parasites. Vaccine. 2010;28:7915–22.
Natarajan R, Thathy V, Mota MM, Hafalla JCR, Ménard R, Vernick KD. Fluorescent Plasmodium berghei sporozoites and pre-erythrocytic stages: a new tool to study mosquito and mammalian host interactions with malaria parasites. Cell Microbiol. 2011;3:371–9.
Frischknecht F, Baldacci P, Martin B, Zimmer C, Thiberge S, Olivo-Marin JC, et al. Imaging movement of malaria parasites during transmission by anopheles mosquitoes. Cell Microbiol. 2004;6:687–94.
Franke-Fayard B, Janse CJ, Cunha-Rodrigues M, Ramesar J, Büscher P, Que I, et al. Murine malaria parasite sequestration: CD36 is the major receptor, but cerebral pathology is unlinked to sequestration. Proc Natl Acad Sci USA. 2005;102:11468–73.
Mizutani M, Fukumoto S, Soubeiga AP, Soga A, Iyori M, Yoshida S. Development of a Plasmodium berghei transgenic parasite expressing the full-length Plasmodium vivax circumsporozoite VK247 protein for testing vaccine efficacy in a murine model. Malar J. 2016;15:251.
O’Donnell RA, de Koning-Ward TF, Burt RA, Bockarie M, Reeder JC, Cowman AF, et al. Antibodies against merozoite surface protein (MSP)-1(19) are a major component of the invasion-inhibitory response in individuals immune to malaria. J Exp Med. 2011;193:1403–12.
Tarun AS, Baer K, Dumpit RF, Gray S, Lejarcegui N, Frevert U, et al. Quantitative isolation and in vivo imaging of malaria parasite liver stages. Int J Parasitol. 2006;36:1283–93.
Labaied M, Harupa A, Dumpit RF, Coppens I, Mikolajczak SA, Kappe SHI. Plasmodium yoelii sporozoites with simultaneous deletion of P52 and P36 are completely attenuated and confer sterile immunity against infection. Infect Immun. 2007;75:3758–68.
Somsak V, Uthaipibull C, Prommana P, Srichairatanakool S, Yuthavong Y, Kamchonwongpaisan S. Transgenic Plasmodium parasites stably expressing Plasmodium vivax dihydrofolate reductase-thymidylate synthase as in vitro and in vivo models for antifolate screening. Malar J. 2011;10:291.
Ozwara H, Langermans JAM, Kocken CHM, Van der Wel A, Van der Meide PH, Vervenne RAW, et al. Transfected Plasmodium knowlesi produces bioactive host gamma interferon: a new perspective for modulating immune responses to malaria parasites. Infect Immun. 2003;71:4375–81.
Serra MP, Carrillo C, González NS, Algranati ID. Modulation of oat arginine decarboxylase gene expression and genome organization in transgenic Trypanosoma cruzi epimastigotes. FEBS J. 2006;273:628–37.
Cayla M, Rachidi N, Leclercq O, Schmidt-Arras D, Rosenqvist H, Wiese M, et al. Transgenic analysis of the Leishmania MAP Kinase MPK10 reveals an auto-inhibitory mechanism crucial for stage-regulated activity and parasite viability. PLoS Pathog. 2014;10:9.
Gay LS, Wilson ME, Donelson JE. The promoter for the ribosomal RNA genes of Leishmania chagasi . Mol Biochem Parasitol. 1996;77:193–200.
Soleimani M, Mahboudi F, Davoudi N, Amanzadeh A, Azizi M, Adeli A, et al. Expression of human tissue plasminogen activator in the trypanosomatid protozoan Leishmania tarentolae . Biotechnol Appl Biochem. 2007;48:55–61.
Banerjee A, Bhattacharya P, Joshi AB, Ismail N, Dey R, Nakhasi HL. Role of pro-inflammatory cytokine IL-17 in Leishmania pathogenesis and in protective immunity by Leishmania vaccines. Cell Immunol. 2016;309:37–41.
Lopez JA, Lebowitz JH, Beverley SM, Rammensee H, Overath P. Leishmania mexicana promastigotes induce cytotoxic T lymphocytes in vivo that do not recognize infected macrophages. Eur J Immunol. 1993;23:217–23.
Pernas L, Adomako-Ankomah Y, Shastri AJ, Ewald SE, Treeck M, Boyle JP, et al. Toxoplasma effector MAF1 mediates recruitment of host mitochondria and impacts the host response. PLoS Biol. 2014;12:4.
Zou J, Huang XX, Yin GW, Ding Y, Liu XY, Wang H, et al. Evaluation of Toxoplasma gondii as a live vaccine vector in susceptible and resistant hosts. Parasit Vectors. 2011;4:168.
Hogquist KA, Jameson SC, Heath WR, Howard JL, Bevan MJ, Carbone FR. T cell receptor antagonist peptides induce positive selection. Cell. 1994;76:17–27.
Barnden MJ, Allison J, Heath WR, Carbone FR. Defective TCR expression in transgenic mice constructed using cDNA-based alpha- and beta-chain genes under the control of heterologous regulatory elements. Immunol Cell Biol. 1998;76:34–40.
Boes M, Cerny J, Massol R, Op den Brouw M, Kirchhausen T, Chen J, et al. T-cell engagement of dendritic cells rapidly rearranges MHC class II transport. Nature. 2002;418:983–8.
Feliu V, Vasseur V, Grover HS, Chu HH, Brown MJ, Wang J, et al. Location of the CD8 T Cell epitope within the antigenic precursor determines immunogenicity and protection against the Toxoplasma gondii parasite. PLoS Pathog. 2013;9:6.
Clark JD, Oakes RD, Redhead K, Crouch CF, Francis MJ, Tomley FM, et al. Eimeria species parasites as novel vaccine delivery vectors: anti- Campylobacter jejuni protective immunity induced by Eimeria tenella -delivered CjaA. Vaccine. 2012;30:2683–8.
Qin M, Liu XY, Tang XM, Suo JX, Tao GR, Suo X. Transfection of Eimeria mitis with yellow fluorescent protein as reporter and the endogenous development of the transgenic parasite. PLoS One. 2014;9:e114188.
Download references
Acknowledgements
We thank Melbourne international scholarship for research training sponsorship.
Author information
Alison L. Every
Present address: College of Science, Health and Engineering, La Trobe University, Melbourne, VIC, 3086, Australia
Authors and Affiliations
Centre for Animal Biotechnology, Faculty of Veterinary and Agricultural Sciences, The University of Melbourne, Melbourne, VIC, 3010, Australia
Mebrahtu G. Tedla, Alison L. Every & Jean-Pierre Y. Scheerlinck
You can also search for this author in PubMed Google Scholar
Contributions
MGT drafted the review paper and it was modified by JYS and ALE. All authors read and approved the final manuscript.
Corresponding author
Correspondence to Mebrahtu G. Tedla .
Ethics declarations
Ethics approval and consent to participate, consent for publication, competing interests.
The authors declare that they have no competing interests.
Additional information
Publisher's note.
Springer Nature remains neutral with regard to jurisdictional claims in published maps and institutional affiliations.
Rights and permissions
Open Access This article is distributed under the terms of the Creative Commons Attribution 4.0 International License ( http://creativecommons.org/licenses/by/4.0/ ), which permits unrestricted use, distribution, and reproduction in any medium, provided you give appropriate credit to the original author(s) and the source, provide a link to the Creative Commons license, and indicate if changes were made. The Creative Commons Public Domain Dedication waiver ( http://creativecommons.org/publicdomain/zero/1.0/ ) applies to the data made available in this article, unless otherwise stated.
Reprints and permissions
About this article
Cite this article.
Tedla, M.G., Every, A.L. & Scheerlinck, JP.Y. Investigating immune responses to parasites using transgenesis. Parasites Vectors 12 , 303 (2019). https://doi.org/10.1186/s13071-019-3550-4
Download citation
Received : 28 January 2019
Accepted : 03 June 2019
Published : 15 June 2019
DOI : https://doi.org/10.1186/s13071-019-3550-4
Share this article
Anyone you share the following link with will be able to read this content:
Sorry, a shareable link is not currently available for this article.
Provided by the Springer Nature SharedIt content-sharing initiative
- Transgenesis
- Immune response
Parasites & Vectors
ISSN: 1756-3305
- Submission enquiries: [email protected]
Thank you for visiting nature.com. You are using a browser version with limited support for CSS. To obtain the best experience, we recommend you use a more up to date browser (or turn off compatibility mode in Internet Explorer). In the meantime, to ensure continued support, we are displaying the site without styles and JavaScript.
- View all journals
Parasite host response articles from across Nature Portfolio
The parasite host response is the process by which a host interacts with and responds to parasites that it encounters. It includes various mechanisms, including immune mechanisms that are elicited in an attempt to eliminate the parasite or halt its growth.
Latest Research and Reviews
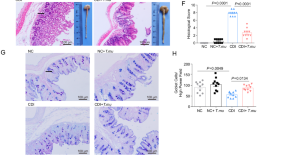

A commensal protozoan attenuates Clostridioides difficile pathogenesis in mice via arginine-ornithine metabolism and host intestinal immune response
Faecal microbiome transplant has been shown to be able to reduce Clostridioides difficile infection. Here the authors show that an intestinal commensal protozoan reduces C. difficile infection by inhibiting neutrophil recruitment and affecting arginine-ornithine metabolism.
- Xiaoxiao Wu
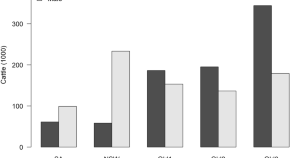
The effect of cystic echinococcosis (hydatid disease) on carcase weight in cattle in eastern Australia
- Victoria J. Brookes
- Tamsin S. Barnes
- Cara S. Wilson
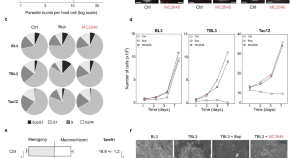
Theileria parasites sequester host eIF5A to escape elimination by host-mediated autophagy
Theileria parasites have evolved mechanisms to evade host cell defenses. Here, Villares et al use an anti-parasite drug to show how intracellular parasites sequester host eIF5A to escape elimination by autophagy pathways.
- Marie Villares
- Nelly Lourenço
- Jonathan B. Weitzman
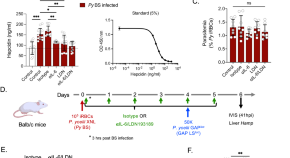
Malaria blood stage infection suppresses liver stage infection via host-induced interferons but not hepcidin
Blood and liver stages of malaria parasites can affect each other, but it’s not clear how this may affect live-attenuated whole parasite vaccination. Here the authors show that malaria parasite blood stage infection subdues new infection and vaccination by suppressing growth of its liver stage via host cytokines.
- Hardik Patel
- Nana K. Minkah
- Stefan H. I. Kappe
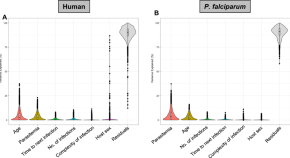
Gene expression analyses reveal differences in children’s response to malaria according to their age
Here the authors use dual RNA sequencing to characterize host and parasite gene expression from 136 Malian children with symptomatic Plasmodium falciparum infection. They find that parasitemia levels correlate with neutrophil and T cell levels and that the child’s age correlates with innate immune gene expression as well as gametocyte levels.
- Kieran Tebben
- Salif Yirampo
- David Serre
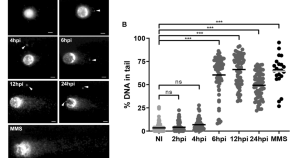
Trypanosoma cruzi infection induces DNA double-strand breaks and activates DNA damage response pathway in host epithelial cells
- Raul Alexander Gonzáles-Córdova
- Thamires Rossi dos Santos
- Munira Muhammad Abdel Baqui
News and Comment
Parasitic control of wolf behaviour.
Meyer et al. show that Toxoplasma gondii infection influences wolf behaviour in the wild, increasing risk-taking behaviours such as dispersing from a pack and becoming a pack leader.
- Michael Attwaters
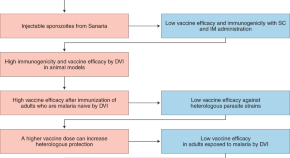
Malaria vaccine roller coaster
A promising vaccine fails to provide durable protection against infection and clinical malaria in infants, a key malaria vaccine target population, in a phase 2b clinical trial. The need for a highly effective vaccine against malaria remains as urgent as ever.
- Irene N. Nkumama
- Faith H. A. Osier
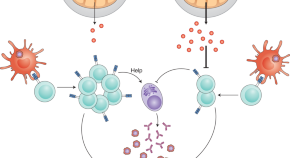
Malaria thriving on steroids
Malaria causes many changes in human metabolism, although the extent to which these changes underpin pathology and the host immune response remains poorly understood. In this issue of Nature Metabolism , Abdrabou et al. show that malaria is associated with elevated levels of circulating steroids in susceptible children and propose that these immunosuppressive lipids exacerbate disease.
- Malcolm J. McConville
- Christian R. Engwerda
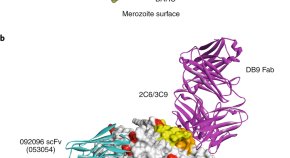
Human antibodies against DBP
Duffy-binding protein (DBP) is the leading vaccine candidate for Plasmodium vivax malaria. Two studies express and characterise the first human monoclonal antibodies against DBP, induced by natural infection and vaccination, showing they have in vitro functional activity but target different conserved epitopes.
- Jack S. Richards
- Paul A. Ramsland
Organoid hosts for parasitic infection
A more natural response.
- Zoltan Fehervari
Quick links
- Explore articles by subject
- Guide to authors
- Editorial policies

Combating Parasites: Immune Response and Inflammation
- First Online: 01 January 2014
Cite this chapter
- David Costantini 2
1413 Accesses
1 Citations
An organism’s physiological equilibrium is critically reliant on its immune system, which provides protection against parasites, pathogens, toxic substances, and cancerous cells and allows recovery from injuries. The immune response is, however, not cost free. It demands various kinds of resources, but also more subtle costs. In the last couple of decades, there has been growing interest by ecophysiologists and evolutionary ecologists in the investigation of oxidative stress as a potential cost of immune activation and induction of anti- and pro-inflammatory mechanisms. A core idea of the immuno-oxidative ecology is that oxidative stress may provide a currency to quantify physiological costs that impinge on growth, reproduction or senescence. This chapter deals with such research and also examines how exposure to mild stress may stimulate activity of immune cells through hormetic mechanisms.
This is a preview of subscription content, log in via an institution to check access.
Access this chapter
- Available as PDF
- Read on any device
- Instant download
- Own it forever
- Available as EPUB and PDF
- Compact, lightweight edition
- Dispatched in 3 to 5 business days
- Free shipping worldwide - see info
- Durable hardcover edition
Tax calculation will be finalised at checkout
Purchases are for personal use only
Institutional subscriptions
Adamo SA (2004) How should behavioural ecologists interpret measurements of immunity? Anim Behav 68:1443–1449
Google Scholar
Almeida BFM, Narciso LG, Melo LM, Preve PP, Bosco AM, Lima VMF, Ciarlini PC (2013) Leishmaniasis causes oxidative stress and alteration of oxidative metabolism and viability of neutrophils in dogs. Veter J 198:599–605
Angelier F, Wingfield JC, Weimerskirch H, Chastel O (2010) Hormonal correlates of individual quality in a long-lived bird: a test of the ‘corticosterone-fitness hypothesis’. Biol Lett 6:846–849
PubMed Central PubMed CAS Google Scholar
Apanius V (1998) Stress and immune defense. Adv Study Behav 27:133–153
Apel K, Hirt H (2004) Reactive oxygen species: metabolism, oxidative stress, and signal transduction. Annu Rev Plant Biol 55:373–399
PubMed CAS Google Scholar
Archie EA, Altmann J, Alberts SC (2012) Social status predicts wound healing in wild baboons. Proc Natl Acad Sci USA 109:9017–9022
Babior BM, Kipnes RS, Curnutte JT (1973) Biological defense mechanisms. The production by leukocytes of superoxide, a potential bactericidal agent. J Clin Invest 52:741–744
Babior BM (1999) NADPH oxidase: an update. Blood 93:1464–1476
CAS Google Scholar
Babior BM (2004) NADPH oxidase. Curr Opin Immunol 16:42–47
Bailey M, Engler H, Hunzeker J, Sheridan JF (2003) The hypothalamic-pituitary-adrenal axis and viral infection. Viral Immunol 16:141–157
Baumann L (1989) The capacity of Ureaplasma urealyticum , Mycoplasma hominis and seven other Mycoplasma species for hemadsorption, sperm adsorption, hemolysis and peroxide formation. Arch Exp Vetmed 43:789–800
Beck MA (1997) Rapid genomic evolution of a non-virulent coxsackievirus B3 in mice due to vitamin E or selenium-deficient mice. Biomed Environ Sci 10:307–315
Bendich A (1989) Carotenoids and the immune response. J Nutr 119:112–115
Bilbo SD, Nelson RJ (2001) Sex steroid hormones enhance immune function in male and female Siberian hamsters. Am J Physiol Regul Integr Comp Physiol 280:207–213
Bjelakovic G, Stojanovic I, Stoimenov TJ, Pavlovic D, Kocic G, Rossi S, Tabolacci C, Nikolic J, Sokolovic D, Bjelakovic L (2010) Metabolic correlations of glucocorticoids and polyamines in inflammation and apoptosis. Amino Acids 39:29–43
Blount JD, Metcalfe NB, Birkhead TR, Surai PF (2003) Carotenoid modulation of immune function and sexual attractiveness in zebra finches. Science 300:125–127
Bogdan C (2007) Oxidative burst without phagocytes: the role of respiratory proteins. Nat Immunol 8:1029–1031
Boleza KA, Burnett LE, Burnett KG (2001) Hypercapnic hypoxia compromises bactericidal activity of fish anterior kidney cells against opportunistic environmental pathogens. Fish Shellfish Immunol 11:593–610
Bonier F, Martin PR, Moore IT, Wingfield JC (2009) Do baseline glucocorticoids predict fitness? Trends Ecol Evol 24:634–642
PubMed Google Scholar
Brecchia G, Cardinali R, Mourvaki E, Collodel G, Moretti E, Dal Bosco A, Castellini C (2010) Short- and long-term effects of lipopolysaccharide-induced inflammation on rabbit sperm quality. Anim Reprod Sci 118:310–316
Broeg K, Steinhagen D (2012) Attack and defense: reactive oxygen and nitrogen species in teleost fish immune response and the coevolved evasion of microbes and parasites. In: Abele D, Vázquez-Medina JP, Zenteno-Savín T (eds) Oxidative stress in aquatic ecosystems. Wiley-Blackwell, UK, pp 247–260
Brousseau P, Pellerin J, Morin Y, Cyr D, Blakley B, Boermans H, Fournier M (2000) Flow cytometry as a tool to monitor the disturbance of phagocytosis in the clam Mya arenaria hemocytes following in vitro exposure to heavy metals. Toxicology 142:145–156
Brune K, Leffell MS, Spitznagel JK (1972) Microbicidal activity of peroxidaseless chicken heterophile leukocytes. Infect Immun 5:283–287
Cadenas S, Rojas C, Barja G (1998) Endotoxin increases oxidative injury to proteins in Guinea pig liver: protection by dietary vitamin C. Pharmacol Toxicol 82:11–18
Calabrese EJ (2005) Hormetic dose-response relationships in immunology: occurrence, quantitative features of the dose response, mechanistic foundations, and clinical implications. Crit Rev Toxic 35:89–295
Calabrese EJ (2013) Historical foundations of wound healing and its potential for acceleration: dose-response considerations. Wound Rep Reg 21:180–193
Carter AW, DuRant SE, Hepp GR, Hopkins WA (2013) Thermal challenge severity differentially influences wound healing in Wood Duck ( Aix sponsa ) ducklings. J Exp Zool 319:422–429
Casagrande S, Costantini D, Groothuis TGG (2012) Interaction between sexual steroids and immune response in affecting oxidative status of birds. Comp Biochem Physiol Part A 163:296–301
Catoni C, Schaffer HM, Peters A (2008) Fruit for health: the effect of flavonoids on humoral immune response and food selection in a frugivorous bird. Funct Ecol 22:649–654
Cerenius L, Lee BL, Soderhall K (2008) The proPO-system: pros and cons for its role in invertebrate immunity. Trends Immuol 29:263–271
Chapman CA, Wasserman MD, Gillespie TR, Speirs ML, Lawes MJ, Saj TL, Ziegler TE (2006) Do food availability, parasitism, and stress have synergistic effects on red colobus populations living in forest fragments? Am J Phys Anthropol 131:525–534
Chew BP (1993) Role of carotenoids in the immune response. J Dairy Sci 76:2804–2811
Christie P, Glaizot O, Strepparava N, Devevey G, Fumagalli L (2012) Twofold cost of reproduction: an increase in parental effort leads to higher malarial parasitaemia and to a decrease in resistance to oxidative stress. Proc R Soc Lond B 279:1142–1149
Cichoń M, Sendecka J, Gustafsson L (2003) Age-related decline in humoral immune function in Collared Flycatchers. J Evol Biol 16:1205–1210
Colic M, Cupic V, Pavicic L, Vucevic D, Varagic VM (2000) Xylazine, and α 2 -adrenergic agonist, modulates proliferation of rat thymocytes in vivo and in vitro. Methods Find Exp Clin Pharmacol 22:557–562
Conlon P, Smith D, Gowlett T (1991) Oxygen radical production by avian leukocytes. Can J Vet Res 55:193–195
Costantini D, Dell’Omo G (2006) Effects of T-cell-mediated immune response on avian oxidative stress. Comp Biochem Physiol Part A 145:137–142
Costantini D, Coluzza C, Fanfani A, Dell’Omo G (2007) Effects of carotenoid supplementation on colour expression, oxidative stress and body mass in rehabilitated captive adult kestrels ( Falco tinnunculus ). J Comp Physiol B 177:723–731
Costantini D, Møller AP (2008) Carotenoids are minor antioxidants for birds. Funct Ecol 22:367–370
Costantini D, Møller AP (2009) Does immune response cause oxidative stress? A meta-analysis. Comp Biochem Physiol Part A 153:339–344
Costantini D, Marasco V, Møller AP (2011) A meta-analysis of glucocorticoids as modulators of oxidative stress in vertebrates. J Comp Physiol B 181:447–456
Cupic V, Colic M, Pavicic L, Vucevic D, Varagic VM (2001) Immunomodulatory effect of xylazine, and α 2 -adrenergic agonist, on rat spleen cells in culture. J Neuroimmunol 113:19–29
Dantzer R, O’Connor JC, Freund GG, Johnson RW, Kelley KW (2008) From inflammation to sickness and depression: when the immune system subjugates the brain. Nat Rev Neurosci 9:46–57
Da Silva CCA (2002) Activation of prophenoloxidase and removal of Bacillus subtilis from the hemolymph of Acheta domesticus L. Orthoptera: Gryllidae. Neotrop Entomol 31:487–491
De Coster G, De Neve L, Martín-Gálvez D, Therry L, Lens L (2010) Variation in innate immunity in relation to ectoparasite load, age and season: a field experiment in great tits ( Parus major ). J Exp Biol 231:3012–3018
De Coster G, De Neve L, Verhulst S, Lens L (2012) Maternal effects reduce oxidative stress in female nestlings under high parasite load. J Avian Biol 43:177–185
De Groote MA, Ochsner UA, Shiloh MU, Nathan C, McCord JM, Dinauer MC, Libby SJ, Vazquez-Torres A, Xu Y, Fang FC (1997) Periplasmic superoxide dismutase protects Salmonella from products of phagocyte NADPH-oxidase and nitric oxide synthase. Proc Natl Acad Sci USA 94:13997–14001
PubMed Central PubMed Google Scholar
De Luca C, Kharaeva Z, Raskovic D, Pastore P, Luci A, Korkina L (2012) Coenzyme Q10, vitamin E, selenium, and methionine in the treatment of chronic recurrent viral mucocutaneous infections. Nutrition 28:509–514
Demas GE, Chefer V, Talan MI, Nelson RJ (1997) Metabolic costs of mounting an antigen-stimulated immune response in adult and aged C57BL/6 J mice. Am J Physiol 273:R1631–R1637
Donaghy L, Lambert C, Choi K-S, Soudant P (2009) Hemocytes of the carpet shell clam ( Ruditapes decussatus ) and the Manila clam ( Ruditapes philippinarum ): current knowledge and future prospects. Aquaculture 297:10–24
Dukan S, Touati D (1996) Hypochlorous acid stress in Escherichia coli : resistance, DNA damage, and comparison with hydrogen peroxide stress. J Bacteriol 178:6145–6150
Elftman MD, Hunzeker JT, Mellinger JC, Bonneau RH, Norbury CC, Truckenmiller ME (2010) Stress-induced glucocorticoids at the earliest stages of herpes simplex virus-1 infection suppress subsequent antiviral immunity, implicating impaired dendritic cell function. J Immunol 184:1867–1875
Faith SA, Sweet TJ, Bailey E, Booth T, Docherty JJ (2006) Resveratrol suppresses nuclear factor-κB in herpes simplex virus infected cells. Antiviral Res 72:242–251
Falchetti R, Fuggetta MP, Lanzilli G, Tricarico M, Ravagnan G (2001) Effects of resveratrol on human immune cell function. Life Sci 70:81–96
Finotello R, Pasquini A, Meucci V, Lippi I, Rota A, Guidi G, Marchetti V (2014) Redox status evaluation in dogs affected by mast cell tumour. Vet Comp Oncol (in press)
Genard B, Miner P, Nicolas J-L, Moraga D, Boudry P, Pernet F, Tremblay R (2013) Integrative study of physiological changes associated with bacterial infection in pacific oyster larvae. PLoS ONE 8:e64534
Georgieva NV, Koinarski V, Gadjeva V (2006) Antioxidant status during the course of Eimeria tenella infection in broiler chickens. Vet J 172:488–492
Gobert AP, McGee DJ, Akhtar M, Mendz GL, Newton JC, Cheng Y, Mobley HLT, Wilson KT (2001) Helicobacter pylori arginase inhibits nitric oxide production by eukaryotic cells: a strategy for bacterial survival. Proc Natl Acad Sci USA 98:13844–13849
Goutte A, Angelier F, Welcker J, Moe B, Clément-Chastel C, Gabrielsen GW, Bech C, Chastel O (2010) Long-term survival effect of corticosterone manipulation in black-legged kittiwakes. Gen Comp Endocr 167:246–251
Gore AB, Qureshi MA (1997) Enhancement of humoral and cellular immunity by vitamin E after embryonic exposure. Poult Sci 76:984–991
Grossman CJ (1985) Interactions between the gonadal steroids and the immune system. Science 227:257–261
Gruver AL, Hudson LL, Sempowski GD (2007) Immunosenescence of ageing. J Pathol 211:144–156
Hall MD, Vettiger A, Ebert D (2013) Interactions between environmental stressors: the influence of salinity on host-parasite interactions between Daphnia magna and Pasteuria ramosa . Oecologia 171:789–796
Halliwell BH (2006) Phagocyte-derived reactive species: salvation or suicide? Trends Biochem Sci 31:509–515
Halliwell BH, Gutteridge JMC (2007) Free radicals in biology and medicine, 4th edn. Oxford University Press, Oxford
Hampton MB, Kettle AJ, Winterboum CC (1998) Inside the neutrophil phagosome: oxidants, myeloperoxidase, and bacterial killing. Blood 92:3007–3017
Harmon BG (1998) Avian heterophils in inflammation and disease resistance. Poult Sci 77:972–977
Hartley RC, Kennedy MW (2004) Are carotenoids a red herring in sexual display? Trends Ecol Evol 19:353–354
Hassan HM, Fridovich I (1979) Paraquat and Escherichia coli . Mechanism of production of extracellular superoxide radical. J Biol Chem 254:10846–10852
Hasselquist D, Nilsson JÅ (2012) Physiological mechanisms mediating costs of immune responses: what can we learn from studies of birds? Anim Behav 83:1303–1312
Heath JJ, Wells B, Cipollini D, Stireman JO III (2013) Carnivores and carotenoids are associated with adaptive behavioural divergence in a radiation of gall midges. Ecol Entomol 38:11–22
Heeb P, Werner I, Kölliker M, Richner H (1998) Benefits of induced host responses against an ectoparasite. Proc R Soc Lond B 265:51–56
Hégaret H, Wikfors GH, Soudant P (2003) Flow cytometric analysis of haemocytes from eastern oysters, Crassostrea virginica , subjected to a sudden temperature elevation: II. Haemocyte functions: aggregation, viability, phagocytosis, and respiratory burst. J Exp Mar Biol Ecol 293:249–265
Hernández JA, Rubio M, Olmos E, Ros-Barceló A, Martínez-Gómez P (2004) Oxidative stress induced by long-term plum pox virus infection in Peach ( Prunus persica ). Physiol Plant 122:486–495
Hoogenboom MO, Metcalfe NB, Groothuis TGG, de Vries B, Costantini D (2012) Relationship between oxidative stress and circulating testosterone and cortisol in pre-spawning female brown trout. Comp Biochem Physiol Part A 163:379–387
Hõrak P, Saks L, Zilmer M, Karu U, Zilmer K (2007) Do dietary antioxidants alleviate the cost of immune activation? An experiment with greenfinches. Am Nat 170:625–635
Hornef MW, Wick MJ, Rhen M, Normark S (2002) Bacterial strategies for overcoming host innate and adaptive immune responses. Nat Immunol 3:1033–1040
Huggins KA, Navara KJ, Mendonça MT, Hill GE (2010) Detrimental effects of carotenoid pigments: the dark side of bright coloration. Naturwissenschaften 97:637–644
Hunzeker JT, Elftman MD, Mellinger JC, Princiotta MF, Bonneau RH, Truckenmiller ME, Norbury CC (2011) A marked reduction in priming of cytotoxic CD8+ T cells mediated by stress-induced glucocorticoids involves multiple deficiencies in cross-presentation by dendritic cells. J Immunol 186:183–194
Isaksson C, Sepil I, Baramidze V, Sheldon BC (2013) Explaining variance of avian malaria infection in the wild: the importance of host density, habitat, individual life-history and oxidative stress. BMC Ecol 13:15
Iyer GYN, Islam DMF, Quastel JH (1961) Biochemical aspects of phagocytosis. Nature 192:535–542
Kaleta EF (1990) Herpesviruses of birds—a review. Avian Pathol 19:193–211
Kavouras JH, Prandovszky E, Valyi-Nagy K, Krisztian Kovacs S, Tiwari V, Kovacs M, Shukla D, Valyi-Nagy T (2007) Herpes simplex virus type 1 infection induces oxidative stress and the release of bioactive lipid peroxidation by-products in mouse P19N neural cell cultures. J Neurovirol 13:416–425
Keil D, Luebke RW, Pruett SB (2001) Quantifying the relationship between multiple immunological and host resistance: probing the limits of reductionism. J Immunol 167:4543–4552
Kenny JF, Pangburn PC, Trail G (1976) Effect of estradiol on immune competence: in vivo and in vitro studies. Infec Immunol 13:448–456
Kim HW, Chew BP, Wong TS, Park JS, Weng BBC, Byrne KM, Hayek MG, Reinhart GA (2000) Modulation of humoral and cell-mediated immune responses by dietary lutein in cats. Vet Immunol Immunopathol 73:331–341
Kitaysky AS, Piatt JF, Wingfield JC (2007) Stress hormones link food availability and population processes in seabirds. Mar Ecol Prog Ser 352:245–258
Kitaysky AS, Piatt JF, Hatch SA, Kitaiskaia EV, Benowitz-Fredericks ZM, Shultz MT, Wingfield JC (2010) Food availability and population processes: severity of nutritional stress during reproduction predicts survival of long lived seabirds. Funct Ecol 24:625–637
Klasing KC (2004) The costs of immunity. Acta Zool Sin 50:961–969
Klasing KC (2007) Nutrition and the immune system. Br Poult Sci 48:525–537
Knowles SCL, Palinauskas V, Sheldon BC (2010) Chronic malaria infections increase family inequalities and reduce parental fitness: experimental evidence from wild bird population. J Evol Biol 23:557–569
Koinarski V, Georgieva N, Gadjeva V, Petkov P (2005) Antioxidant status of broiler chickens, infected with Eimeria acervulina . Revue Méd Vét 156:498–502
Krams I, Daukšte J, Kivleniece I, Kaasik A, Krama T, Freeberg TM, Rantala MJ (2013) Trade-off between cellular immunity and life span in meal-worm beetles Tenebrio molitor . Curr Zool 59:340–346
Kurhalyuk N, Tkachenko H, Pałczyńska K (2009) Antioxidant enzymes profile in the brown trout ( Salmo trutta trutta ) with ulcerative dermal necrosis. Bull Vet Inst Pulawy 53:813–818
Kurhalyuk N, Tkachenko H, Palczyńska K (2010) Lipid peroxidation and antioxidant defense system in spawn of brown trout ( Salmo trutta m. trutta L.) affected by ulcerative dermal necrosis. Arch Pol Fish 18:115–122
Kurtz J, Wegner MK, Kalbe M, Reusch TBH, Schaschl H, Hasselquist D, Milinski M (2006) MHC genes and oxidative stress in sticklebacks: an immuno-ecological approach. Proc R Soc Lond B 273:1407–1414
Kurtz J, Kalbe M, Langefors Å, Mayer I, Milinski M, Hasselquist D (2007) An experimental test of the immunocompetence handicap hypothesis in a teleost fish: 11-ketotestosterone suppresses innate immunity in three-spined sticklebacks. Am Nat 170:509–519
Lambert C, Nicolas JL (1998) Specific inhibition of chemiluminescent activity by pathogenic vibrios in hemocytes of two marine bivalves: Pecten maximus and Crassostrea gigas . J Invert Pathol 71:53–63
Leroy M, Mosser T, Manière X, Fernández Alvarez D, Matic I (2012) Pathogen-induced Caenorhabditis elegans developmental plasticity has a hormetic effect on the resistance to biotic and abiotic stresses. BMC Evol Biol 12:187
Levy O (2004) Antimicrobial proteins and peptides: anti-infective molecules of mammalian leukocytes. J Leukoc Biol 76:909–925
Liu GY, Essex A, Buchanan JT, Datta V, Hoffman HM, Bastian JF, Fierer J, Nizet V (2005) Staphylococcus aureus golden pigment impairs neutrophil killing and promotes virulence through its antioxidant activity. J Exp Med 202:209–215
Los M, Droge W, Stricker K, Baeuerle PA, Schulze-Osthoff K (1995) Hydrogen peroxide as a potent activator of T lymphocyte functions. Eur J Immunol 15:159–165
Losdat S, Richner H, Blount JD, Helfenstein F (2011) Immune activation reduces sperm quality in the great tit. PLoS ONE 6:e22221
Lowder T, Padgett DA, Woods JA (2005) Moderate exercise protects mice from death due to influenza virus. Brain Behav Immunol 19:377–380
Lynch RE, Cole BC (1980) Mycoplasma pneumoniae : a prokaryote which consumes oxygen and generates superoxide but which lacks superoxide dismutase. Biochem Biophys Res Commun 96:98–105
Martin LB, Hopkins WA, Mydlarz LD, Rohr JR (2010) The effects of anthropogenic global changes on immune functions and disease resistance. Ann NY Acad Sci 1195:129–148
Martin LB, Hawley DM, Ardia DR (2011) An introduction to ecological immunology. Funct Ecol 25:1–4
Mastroeni P, Vazquez-Torres A, Fang FC, Xu Y, Khan S, Hormaeche CE, Dougan G (2000) Antimicrobial actions of the NADPH phagocyte oxidase and inducible nitric oxide synthase in experimental salmonellosis. II. Effects on microbial proliferation and host survival in vivo . J Exp Med 192:237–247
Mathew SS, Bryant PW, Burch AD (2010) Accumulation of oxidized proteins in Herpesvirus infected cells. Free Rad Biol Med 49:383–391
McEwen BS, Stellar E (1993) Stress and the individual—mechanisms leading to disease. Arch Inter Med 153:2093–2101
McGraw KJ, Ardia DR (2003) Carotenoids, immunocompetence, and the information content of sexual colors: an experimental test. Am Nat 162:704–712
Meitern R, Sild E, Lind M-A, Männiste M, Sepp T, Karu U, Hõrak P (2013) Effects of endotoxin and psychological stress on redox physiology, immunity and feather corticosterone in greenfinches. PLoS ONE 8:e67545
Mougeot F, Martínez-Padilla J, Webster LMI, Blount JD, Pérez-Rodríguez L, Piertney SB (2009) Honest sexual signalling mediated by parasite and testosterone effects on oxidative balance. Proc R Soc Lond B 276:1093–1100
Munck A, Guyre PM, Holbrook NJ (1984) Physiological functions of glucocorticoids in stress and their relation to pharmacological actions. Endocr Rev 5:25–44
Murphy EA, Davis JM, Carmichael MD, Gangemi JD, Ghaffar A, Mayer EP (2008) Exercise stress increases susceptibility to influenza infection. Brain Behav Immunol 22:1152–1155
Nathan C, Shiloh MU (2000) Reactive oxygen and nitrogen intermediates in the relationship between mammalian hosts and microbial pathogens. Proc Natl Acad Sci USA 97:8841–8848
Ndong D, Chen Y-Y, Lin Y-H, Vaseeharan B, Chen J-C (2006) The immune response of tilapia Oreochromis mossambicus and its susceptibility to Streptococcus iniae under stress in low and high temperatures. Fish Shellfish Immunol 22:686–694
Norris K, Evans MR (2000) Ecological immunology: life-history trade-offs and immune defense in birds. Behav Ecol 11:19–26
Nussey DH, Pemberton JM, Pilkington JG, Blount JD (2009) Life history correlates of oxidative damage in a free-living mammal population. Funct Ecol 23:809–817
Ots I, Kerimov AB, Ivankina EV, Ilyna TA, Hõrak P (2001) Immune challenge affects basal metabolic activity in wintering great tits. Proc R Soc Lond B 268:1175–1181
Paltrinieri S, Ravicini S, Rossi G, Roura X (2010) Serum concentrations of the derivatives of reactive oxygen metabolites (d-ROMs) in dogs with leishmaniosis. Veter J 186:393–395
Panaro MA, Lisi S, Mitolo V, Acquafredda A, Fasanella A, Carelli MG, Brandonisio O (1998) Evaluation of killing, superoxide anion and nitric oxide production by Leishmania infantum -infected dog monocytes. Cytobios 95:151–160
Pašková V, Adamovský O, Pikula J, Skočovská B, Band’ouchová H, Horáková J, Babica P, Maršálek B, Hilscherová K (2008) Detoxification and oxidative stress responses along with microcystins accumulation in Japanese quail exposed to cyanobacterial biomass. Sci Total Environ 398:34–47
Pedersen AB, Babayan SA (2011) Wild immunology. Mol Ecol 20:872–880
Penniall R, Spitznagel JK (1975) Chicken neutrophils: oxidative metabolism in phagocytic cells devoid of myeloperoxidase. Proc Natl Acad Sci USA 72:5012–5015
Pereira B, Rosa LF, Safi DA, Bechara EJ, Curi R (1995) Hormonal regulation of superoxide dismutase, catalase, and glutathione peroxidase activities in rat macrophages. Biochem Pharmacol 50:2093–2098
Philipp EER, Lipinski S, Rast J, Rosenstiel P (2012) Immune defense of marine invertebrates: the role of reactive oxygen and nitrogen species. In: Abele D, Vázquez-Medina JP, Zenteno-Savín T (eds) Oxidative stress in aquatic ecosystems. Wiley-Blackwell, UK, pp 236–246
Pipe RK, Farley SR, Coles JA (1997) The separation and characterisation of haemocytes from the mussel Mytilus edulis . Cell Tissue Res 289:537–545
Pruett SB, Ensley DK, Crittenden PL (1993) The role of chemical-induced stress responses in immunosuppression: a review of quantitative associations and cause-effect relationships between chemical-induced stress responses and immunosuppression. J Toxicol Environ Health 39:163–192
Qureshi MA, Heggen CL, Hussain I (2000) Avian macrophage: effector functions in health and disease. Devel Comp Immunol 24:103–119
Rajan TV, Porte P, Yates JA, Keefer L, Shultz LD (1996) Role of nitric oxide in host defense against an extracellular, metazoan parasite, Brugia malayi . Infect Immun 64:3351–3353
Reddy MM, Mahipal SVK, Subhashini J, Reddy MC, Roy KR, Reddy GV, Reddy PR, Reddanna P (2006) Bacterial lipopolysaccharide-induced oxidative stress in the impairment of steroidogenesis and spermatogenesis in rats. Reprod Toxicol 22:493–500
Repine JE, Fox RB, Berger EM (1981) Hydrogen peroxide kills Staphylococcus aureus by reacting with staphylococcal iron to form hydroxyl radical. J Biol Chem 256:7094–7096
Ridgway I, Bowden TJ, Roman-Gonzalez A, Richardson CA (2014) Resistance to oxidative stress is not associated with the exceptional longevity of the freshwater pearl mussel, Margaritifera margaritifera nor three unionid species. Aquat Sci (in press)
Riedel W, Maulik G (1999) Fever: an integrated response of the central nervous system to oxidative stress. Mol Cell Biochem 196:125–132
Romero LM, Wikelski M (2001) Corticosterone levels predict survival probabilities of Galapagos marine iguanas during El Niño events. Proc Natl Acad Sci USA 98:7366–7370
Romero LM (2004) Physiological stress in ecology: lessons from biomedical research. Trends Ecol Evol 19:249–255
Romero LM, Dickens MJ, Cyr NE (2009) The reactive scope model—a new model integrating homeostasis, allostasis, and stress. Horm Behav 55:375–389
Sacks D, Sher A (2002) Evasion of innate immunity by parasitic protozoa. Nat Immunol 3:1041–1047
Sapolsky RM, Romero LM, Munck AU (2000) How do glucocorticoids influence stress responses? Integrating permissive, suppressive, stimulatory, and preparative actions. Endocr Rev 21:55–89
Schneeberger K, Czirják GA, Voigt CC (2013) Inflammatory challenge increases measures of oxidative stress in a free-ranging, long-lived mammal. J Exp Biol 216:4514–4519
Schulenburg H, Kurtz J, Moret Y, Siva-Jothy MT (2009) Introduction. Ecological immunology. Phil Trans R Soc B 364:3–14
Schwanz LE (2006) Schistosome infection in deer mice ( Peromyscus maniculatus ): impacts on host physiology, behavior and energetics. J Exp Biol 209:5029–5037
Segal AW (2005) How neutrophils kill microbes. Annu Rev Immunol 23:197–223
Sepp T, Karu U, Blount JD, Sild E, Männiste M, Hõrak P (2012) Coccidian infection causes oxidative damage in greenfinches. PLoS ONE 7:e36495
Seve M, Favier A, Osman M, Hernandey B, Vaitaitis G, Flores NC, McCord JM, Flores SC (1999) The human immunodeficiency virus-1-tat protein increases cell proliferation, alters sensitivity to zinc chelator-induced apoptosis, and changes Sp1 DNA binding in Hera cell. Arch Biochem Biophys 36:165–172
Sheldon BC, Verhulst S (1996) Ecological immunology: costly parasite defences and trade-offs in evolutionary ecology. Trends Ecol Evol 11:317–321
Shen Z, Wu W, Hazen SL (2000) Activated leukocytes oxidatively damage DNA, RNA, and the nucleotide pool through halide-dependent formation of hydroxyl radical. Biochemistry 39:5474–5482
Sheridan PA, Beck MA (2008) The immune response to herpes simplex virus encephalitis in mice is modulated by dietary vitamin E. J Nutr 138:130–137
Shi L, Sohaskey CD, Kana BD, Dawes S, North RJ, Mizrahi V, Gennaro ML (2005) Changes in energy metabolism of Mycobacterium tuberculosis in mouse lung and under in vitro conditions affecting aerobic respiration. Proc Natl Acad Sci USA 102:15629–15634
Shukitt-Hale B, Galli RL, Meterko V, Carey A, Bielinski DF, McGhie T, Joseph JA (2005) Dietary supplementation with fruit polyphenolics ameliorates age-related deficits in behavior and neuronal markers of inflammation and oxidative stress. Age 27:49–57
Sild E, Sepp T, Männiste M, Hõrak P (2011) Carotenoid intake does not affect immune-stimulated oxidative burst in greenfinches. J Exp Biol 214:3467–3473
Simons MJP, Cohen AA, Verhulst S (2012) What does carotenoid-dependent coloration tell? Plasma carotenoid level signals immunocompetence and oxidative stress state in birds–a meta-analysis. PLoS ONE 7:e43088
Sirak AA, Beavis AJ, Robertson FM (1991) Enhanced hydroperoxide production by peripheral blood leukocytes following exposure of murine epidermis to 12-Otetradecanoylphorbol-13-acetate. Carcinogenesis 12:91–95
Sorci G, Faivre B (2009) Inflammation and oxidative stress in vertebrate host–parasite systems. Philos Trans Roy Soc B 364:71–83
Stabler JG, McCormick TW, Powell KC, Kogut MH (1994) Avian heterophils and monocytes: phagocytic and bactericidal activities against Salmonella enteritidis . Vet Microbiol 38:293–305
Stanulis ED, Jordan SD, Rosecrans JA, Holsapple MP (1997a) Disruption of Th1/Th2 cytokine balance by cocaine is mediated by corticosterone. Immunopharmacology 37:25–33
Stanulis ED, Matulka RA, Jordan SD, Rosecrans JA, Holsapple MP (1997b) Role of corticosterone in the enhancement of the antibody response after acute cocaine administration. J Pharmacol Exp Ther 280:284–291
Surai PF (2002) Natural antioxidants in avian nutrition and reproduction. Nottingham University Press, Nottingham
Tobler M, Healey M, Wilson M, Olsson M (2011) Basal superoxide as a sex-specific immune constraint. Biol Lett 7:906–908
Torres R, Velando A (2007) Male reproductive senescence: the price of immune-induced oxidative damage on sexual attractiveness in the blue-footed booby. J Anim Ecol 76:1161–1168
Tschirren B, Fitze PS, Richner H (2003) Sexual dimorphism in susceptibility to parasites and cell-mediated immunity in great tit nestlings. J Anim Ecol 72:839–845
Üllen A, Singewald E, Konya V, Fauler G, Reicher H, Nusshold C, Hammer A, Kratky D, Heinemann A, Holzer P, Malle E, Sattler W (2013) Myeloperoxidase-derived oxidants induce blood-brain barrier dysfunction in vitro and in vivo . PLoS ONE 8:e64034
van de Crommenacker J, Horrocks NPC, Versteegh MA, Komdeur J, Tieleman BI, Matson KD (2010) Effects of immune supplementation and immune challenge on oxidative status and physiology in a model bird: implications for ecologists. J Exp Biol 213:3527–3535
van de Crommenacker J, Richardson DS, Koltz AM, Hutchings K, Komdeur J (2012) Parasitic infection and oxidative stress are associated and vary with breeding activity in the Seychelles warbler. Proc R Soc Lond B 279:1466–1476
Vilchèze C, Hartman T, Weinrick B, Jacobs WR Jr (2013) Mycobacterium tuberculosis is extraordinarily sensitive to killing by a vitamin C-induced Fenton reaction. Nat Comm 4:1881
Webster JI, Tonelli L, Sternberg EM (2002) Neuroendocrine regulation of immunity. Annu Rev Immunol 20:125–163
Whitfield JF, MacManus JP, Rixon RH (1970) Cyclic AMP mediated stimulation of thymocyte proliferation by low concentrations of cortisol (34967). Proc Soc Exp Biol Med 134:1170–1174
Wingfield JC, Maney DL, Breuner CW, Jacobs JD, Lynn S, Ramenofsky M, Richardson RD (1998) Ecological bases of hormone-behavior interactions: the “emergency life history stage”. Am Zool 38:191–206
Winter JL, Barber LG, Freeman L, Griessmayr PC, Milbury PE, Blumberg JB (2009) Antioxidant status and biomarkers of oxidative stress in dogs with lymphoma. J Vet Intern Med 23:311–316
Winterbourn CC (2002) Biological reactivity and biomarkers of the neutrophil oxidant, hypochlorous acid. Toxicology 181(182):223–227
Wood MJ, Cosgrove CL, Wilkin TA, Knowles SCL, Day KP, Sheldon BC (2007) Within-population variation in prevalence and lineage distribution of avian malaria in blue tits, Cyanistes caeruleus . Mol Ecol 16:3263–3273
Woods JA, Davis JM, Smith JA, Nieman DC (1999) Exercise and cellular innate immune function. Med Sci Sports Exerc 31:57–66
Xu J, Yin Z, Li L, Cheng A, Jia R, Song X, Lu H, Dai S, Lv C, Liang X, He C, Zhao L, Su G, Ye G, Shi F (2013) Inhibitory effect of resveratrol against duck enteritis virus in vitro . PLoS ONE 8:e65213
Zahran E, Risha E (2013) Protective role of adjuvant and potassium permanganate on oxidative stress response of Nile tilapia ( Oreochromis niloticus ) challenged with Saprolegnia ferax . Springerplus 2:94
Download references
Author information
Authors and affiliations.
Department of Biology, University of Antwerp, Universiteitsplein House No. 1, 2610, Wilrijk, Antwerp, Belgium
David Costantini
You can also search for this author in PubMed Google Scholar
Corresponding author
Correspondence to David Costantini .
Rights and permissions
Reprints and permissions
Copyright information
© 2014 Springer-Verlag Berlin Heidelberg
About this chapter
Costantini, D. (2014). Combating Parasites: Immune Response and Inflammation. In: Oxidative Stress and Hormesis in Evolutionary Ecology and Physiology. Springer, Berlin, Heidelberg. https://doi.org/10.1007/978-3-642-54663-1_8
Download citation
DOI : https://doi.org/10.1007/978-3-642-54663-1_8
Published : 28 March 2014
Publisher Name : Springer, Berlin, Heidelberg
Print ISBN : 978-3-642-54662-4
Online ISBN : 978-3-642-54663-1
eBook Packages : Biomedical and Life Sciences Biomedical and Life Sciences (R0)
Share this chapter
Anyone you share the following link with will be able to read this content:
Sorry, a shareable link is not currently available for this article.
Provided by the Springer Nature SharedIt content-sharing initiative
- Publish with us
Policies and ethics
- Find a journal
- Track your research

Helminth Parasites' Regulation of Host Immune Response Reduces Inflammatory Disease Symptoms
New information about how helminths regulate their hosts’ immune system may provide valuable insight for therapies to fight inflammatory diseases.
Helminths (namely, cestode tapeworms, nematode roundworms, and trematode flukes) are long-lived worm parasites that have evolved methods to dampen the host’s immune response, protecting the parasite from elimination by the host, and minimizing severe disease in the host. In a recent review article , in the Journal of Allergy and Clinical Immunology, Rick M. Maizels, PhD, from the University of Glasgow, Scotland, and Henry J. McSorley, from the University of Edinburgh, Scotland, discuss some of immunomodulatory mechanisms associated with helminth infections, how they apply to human disease, and possible areas for future research.
Helminths and the Immune System
Helminths are very successful parasites and their success results from their active regulation of the host immune response. Although only about a dozen helminth species are widespread in humans, together they infect almost one-third of the world’s population—often for up to 20 years. “Their extraordinary prevalence bears witness to their success at defeating host defences,” the authors write. This reflects their ability to manipulate the host’s immune system and dampen responses that could eliminate them. Studies have shown the ability of helminths to induce, modulate, or suppress certain pathways of immune activation in the host.
Induction of T helper (Th) type 2 immune responses with a regulatory component is a classic host response to helminth infections, and dendritic cells play a key role in this induction. Although exactly how these cells recognize the presence of helminths remains unknown, KLF4 factor is an essential intracellular signal that allows dendritic cells to promote Th2 responses.
A critical link has also emerged between helminth infection and increased production of regulatory cells, especially regulatory T cells (Tregs)—including those expressing the transcription factor Foxp3—that block protective immune responses. These cells are essential to allow helminths to survive in the host and avoid being expelled, but evidence shows that they may also protect the host from pathology. Helminth-induced regulatory B cells (Bregs) also play an important role in modulating the immune response during helminth infection. Emerging evidence also suggests that these cells can suppress allergic inflammation. “Along with Treg[s], Bregs are strongly implicated in the development of tolerance to allergens and strategies to encourage their expansion could increase the efficacy of allergen-specific immunotherapy,” the authors write.
Helminths can effectively modulate the adaptive immune response to enable them to survive in the host. For example, they can promote Treg differentiation, either directly by producing a TGF-β-like mimic or indirectly by inducing host TGF-β and retinoic acid (RA) production by dendritic cells and macrophages. This allows the parasites to hijack the host’s TGF-β signaling pathway, suppress the host’s protective Th2 response, and thereby avoid immune attack.
Suppression
Helminths suppress some key pathways of immune activation in the host. For example,
when helminths cause tissue damage during infection, they trigger release of alarmin cytokines, including interleukin 33 which activates innate lymphoid cells that promote development of the type 2 response against the helminths; the parasites also inhibit toll-like receptor (TLR) responses of dendritic cells. However, with repeated infection, the host’s immune responses are dampened: helminths can effectively block alarmin production and TLR function, ultimately preventing the T helper (Th) type 2 immune response.
Allergy, Autoimmunity, and Allograft Rejection
Helminth-induced immunomodulation can be beneficial for the host, as well as the parasite. “It has been noted since 1968 that inflammatory disorders such as arthritis are much less frequent in low-income countries with high levels of parasite infection,” the authors note. And regions of the world where helminth parasites are endemic have a lower prevalence of allergies and autoimmune conditions—as well as increases in allergic reactivity and autoimmune antibodies after anthelmintic treatment. According to the authors, helminths probably affect the immune system at two levels—by modifying the level of host reactivity during development of the immune system during infancy, and by suppressing immune responses in mature individuals who may be exposed to helminths for the first time in adulthood. “It is the latter setting that led to the proposal that helminths or their products could be used as therapies for inflammatory diseases in the parasite-free developed world,” they say. Similarly, evidence is mounting to suggest that helminth infections may protect against allograft rejection in transplant recipients.
Future Directions
The authors also stress that increased understanding of immunosuppressed responses to helminths, and of how to neutralize these responses, may improve understanding of anti-cancer responses. “Parasitic infection could increase carcinogenesis through associated low-grade chronic inflammatory response (in the absence of parasite ejection), secretion of directly pro-carcinogenic factors, or suppression of immune surveillance,” they state. “Epidemiological data in this area is presently lacking, and the effects of parasitic infection in cancer progression requires further attention.
“As we discover more about how productive anti-parasite responses are produced, we are also discovering new pathways for immunomodulation of these pathways by helminth infections, and exploring new possibilities for exploiting parasite molecules as therapies for inflammatory diseases,” the authors conclude.
Dr. Parry graduated from the University of Liverpool, England in 1997 and is a board-certified veterinary pathologist. After 13 years working in academia, she founded Midwest Veterinary Pathology, LLC where she now works as a private consultant. She is passionate about veterinary education and serves on the Indiana Veterinary Medical Association’s Continuing Education Committee. She regularly writes continuing education articles for veterinary organizations and journals, and has also served on the American College of Veterinary Pathologists’ Examination Committee and Education Committee.
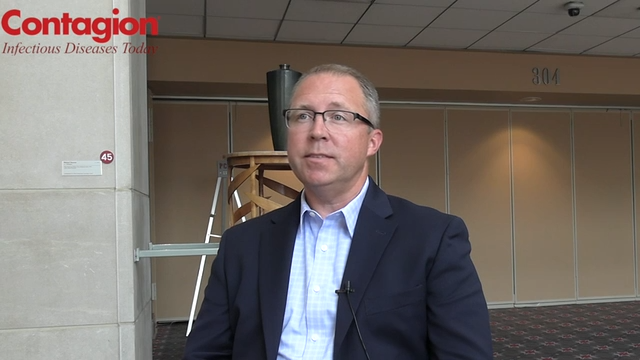
World Malaria Day: Addressing Challenges and Advances in Malaria Research
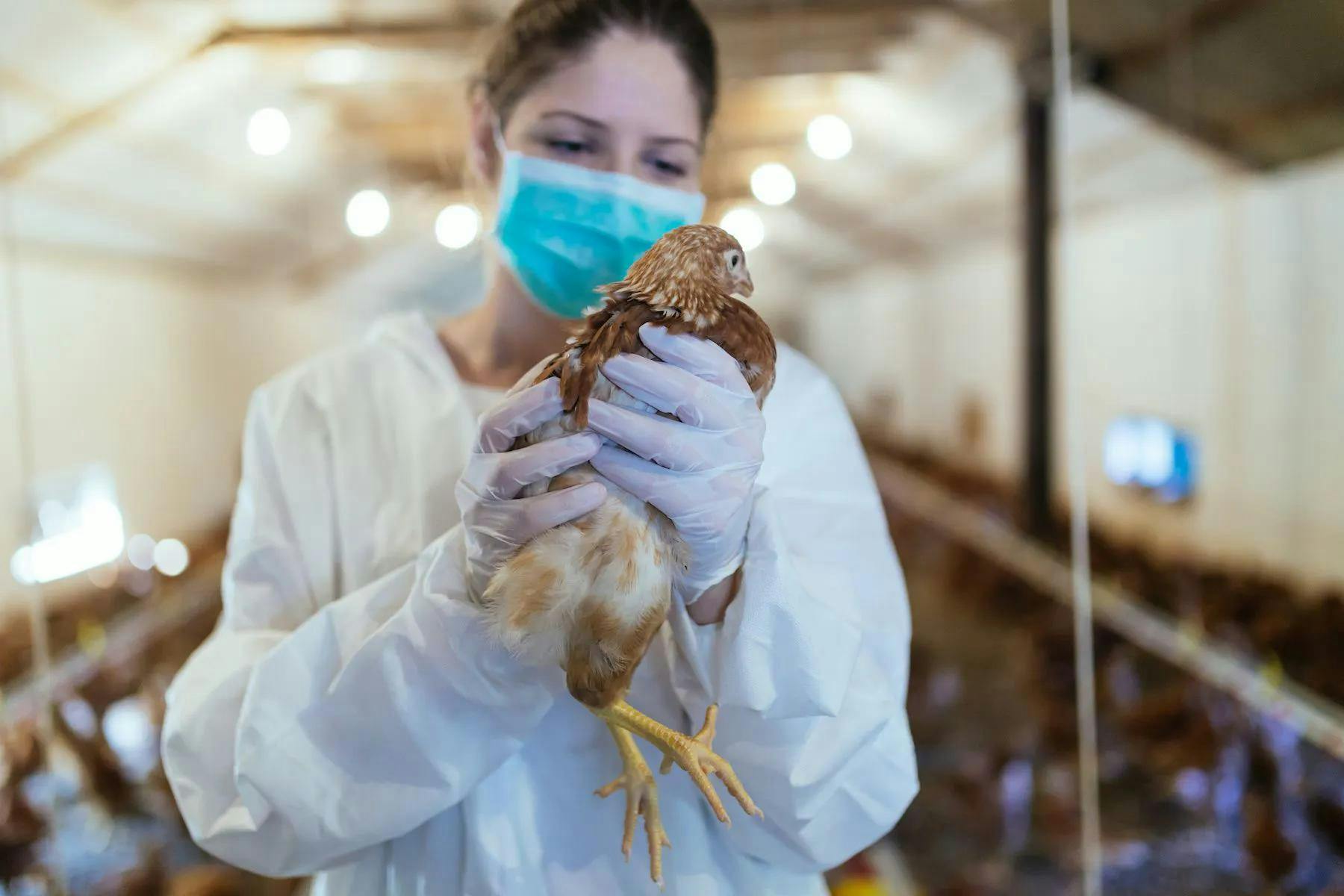
Addressing Public Health Concerns Over Avian Flu
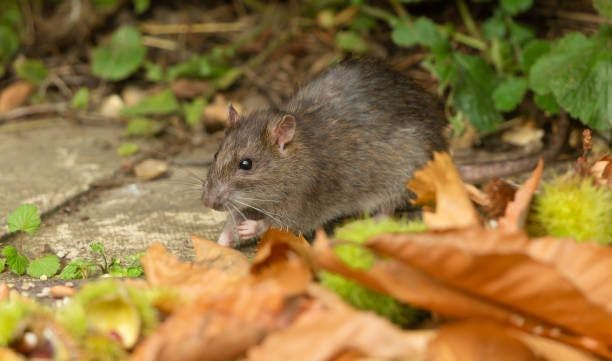
Rise in Leptospirosis Cases in New York City
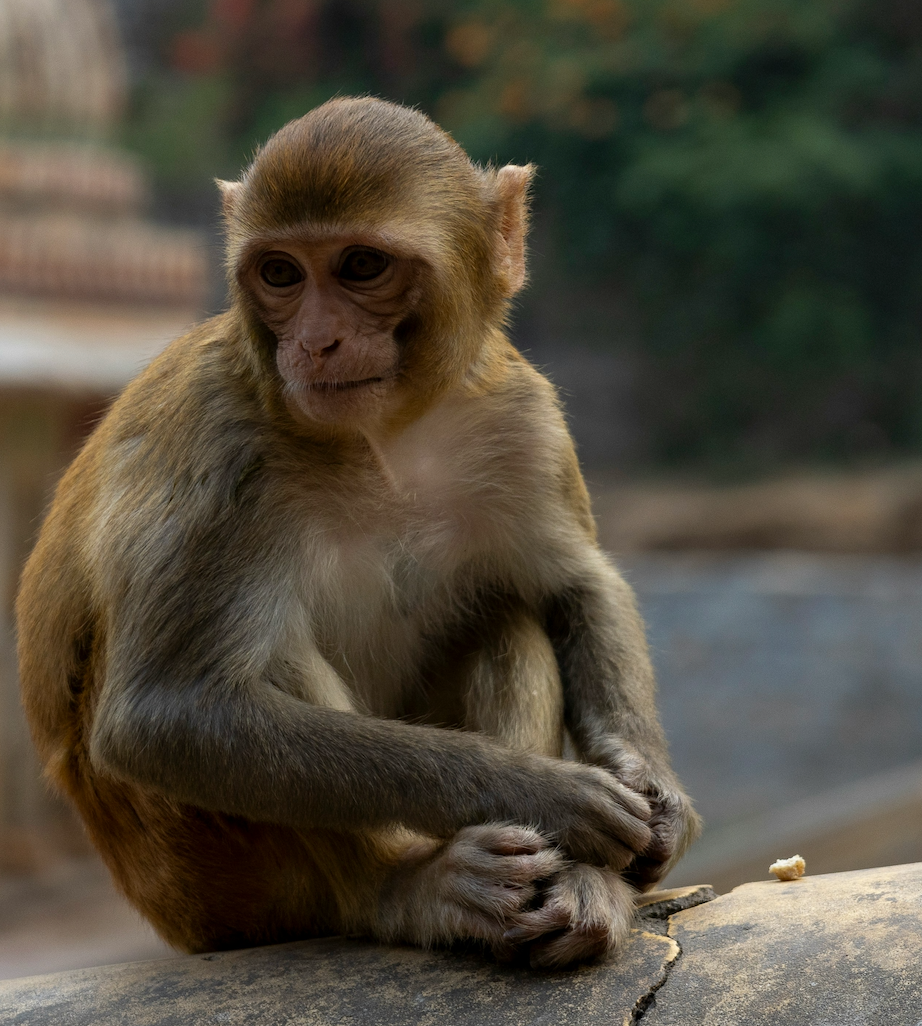
Hong Kong Reports First Human Case of B Virus
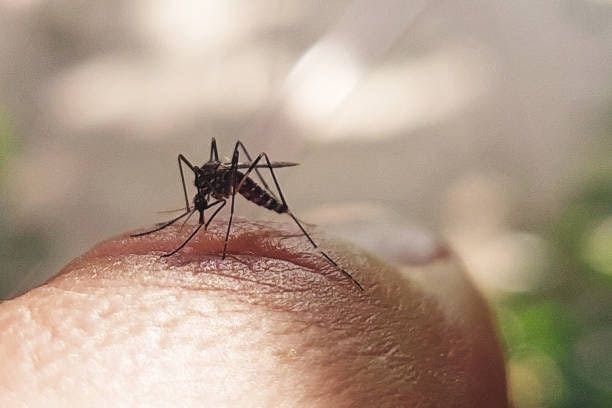
Puerto Rico Declares Public Health Emergency
2 Commerce Drive Cranbury, NJ 08512
609-716-7777


An official website of the United States government
The .gov means it’s official. Federal government websites often end in .gov or .mil. Before sharing sensitive information, make sure you’re on a federal government site.
The site is secure. The https:// ensures that you are connecting to the official website and that any information you provide is encrypted and transmitted securely.
- Publications
- Account settings
Preview improvements coming to the PMC website in October 2024. Learn More or Try it out now .
- Advanced Search
- Journal List
- HHS Author Manuscripts

Immunology of lymphatic filariasis
Subash babu.
1 NIAID-NIRT-ICER Chennai India
Thomas B. Nutman
2 Helminth Immunology Section Laboratory of Parasitic Diseases National Institute of Allergy and Infectious Diseases Bethesda, MD 20892-0425
The immune responses to filarial parasites encompass a complex network of innate and adaptive cells whose interaction with the parasite underlies a spectrum of clinical manifestations. The predominant immunological feature of lymphatic filariasis is an antigen - specific Th2 response and an expansion of IL-10 producing CD4 + T cells that is accompanied by a muted Th1 response. This antigen specific T cell hypo-responsiveness appears to be crucial for the maintenance of the sustained, long-standing infection often with high parasite densities. While the correlates of protective immunity to lymphatic filariasis are still incompletely understood, primarily due to the lack of suitable animal models to study susceptibility, it is clear that T cells and to a certain extent B cells are required for protective immunity. Host immune responses, especially CD4 + T cell responses clearly play a role in mediating pathological manifestations of LF, including lymphedema, hydrocele and elephantiasis. The main underlying defect in the development of clinical pathology appears to be a failure to induce T cell hypo-responsiveness in the face of antigenic stimulation. Finally, another intriguing feature of filarial infections is their propensity to induce bystander effects on a variety of immune responses, including responses to vaccinations, allergens and to other infectious agents. The complexity of the immune response to filarial infection therefore provides an important gateway to understanding the regulation of immune responses to chronic infections, in general.
Introduction
Lymphatic filariasis is an infection caused by three closely related nematode worms – Wuchereria bancrofti, Brugia malayi and Brugia timori . The parasites are transmitted by mosquitoes and their life cycles in humans consists of adult worms living in the afferent lymphatic vessels while their progeny, the microfilariae, circulate in the peripheral blood. Although typically clinically asymptomatic, lymphatic filariasis can also be associated with lymphatic disease, which is responsible for considerable suffering, deformity and disability and is the second leading parasitic cause of disability with DALYs (disability-adjusted life years) estimated to be 5.549 million ( 1 ). Bancroftian filariasis, caused by W. bancrofti is responsible for 90% of those with lymphatic filariasis ( 2 ) and the rest are caused by Brugian parasites. Lymphatic filariasis is a global health problem. At the present time (2013), the World Health Organization estimated that over 1.25 billion people are at risk in 72 countries and territories. It is known that approximately 120 million people infected with lymphatic filariasis and over 40 million have pathologic consequences, including the disfiguring elephantiasis. Clinical disease is manifested primarily as acute adenolymphangitis and chronic lymphedema that may lead to elephantiasis in men and women and to the formation of hydroceles (for W. bancrofti at least) in men.
All human filarial nematodes have a complex life cycle involving an insect vector, with Wuchereria and Brugia being transmitted by mosquitoes. Infection begins with the deposition of infective larvae (L3) in the skin during a mosquito bite. The larvae then enter through the puncture wound, reaching the lymphatics and migrate towards the lymph nodes. They reside within the lymphatics and lymph nodes and undergo a process of molting and development to form L4 larvae and then adult worms. Following mating the adult female releases live progeny called microfilariae that circulate in the bloodstream. These microfilariae can then be ingested by a mosquito during a subsequent blood meal, where in they undergo development to form L2 and finally L3 larvae. The complex life cycle engenders a complicated host immune response, and it is this complexity of the host-parasite interaction that is thought to underlie the varied clinical manifestations of lymphatic filariasis. Lymphatic filariasis can manifest itself in a variety of clinical and subclinical conditions ( 2 ). The most common clinical manifestation is a relatively asymptomatic or subclinical infection characterized by the presence circulating microfilariae and the relative absence of clinical symptoms. A subgroup of individuals present clinically with overt pathology in the form of lymphedema, hydrocele and elephantiasis. In this review, we discuss mostly studies pertaining to human filarial infections and have used the Brugia infection of mice as the primary model for studying host immune interaction with filarial parasites and referred to the Litomosoides model only in the absence of data from the Brugian model.
Prototypical immune responses
The canonical host immune response to filarial parasites in both mice and humans is of the T helper 2 (Th2) type and involves the production of cytokines – IL-4, IL-5, IL-9, IL-10 and IL-13, the antibody isotypes – IgG1, IgG4 (in humans) and IgE, and expanded populations of eosinophils and alternatively activated macrophages ( 3 ). The initial interaction of T cells with a variety of host cell types including dendritic cells and macrophages induces and culminates in Th2 responses ( 3 ). While dendritic cells, basophils and innate lymphoid cells have been all shown to initiate Th2 responses in other helminth infections, their role in the initiation of early Th2 responses in filarial infections is still unclear. Type 2 innate lymphoid cells (ILCs) have been shown to be important players in the initiation of Th2 responses in helminth infections ( 4 , 5 ). Recent data from our lab has shown that ILC2 are expanded in both mouse models and human filarial infections and produce copious amount of IL-5 and IL-13 at times preceding the onset of classical Th2 responses (unpublished results). Thus, ILC2 might be important players in orchestrating the establishment of the Th2 response in filarial infections. These prototypical Th2 responses are modulated by both adaptive and natural regulatory T cells, alternatively activated macrophages, eosinophils and other cell populations over the duration of infection ( 6 ). The main characteristic of a chronic filarial infection appears to be the presence of a modified Th2 response and an IL-10 dominated regulatory environment ( 6 ).
T cells are the key players in immunity to filarial infections. Both nude mice (that lack T cells) ( 7 , 8 ) and SCID or Rag-deficient mice (that lack both T and B cells) ( 9 , 10 ) are susceptible to infection with Brugian parasites, indicating that T cells are absolutely critical for elimination of infection. It appears, however, that either T cell subset - CD4 + or CD8 + T cells - can mediate resistance in non-permissive animals since mice that lack either CD4 + T cells or CD8 + T cell are fully capable of resisting infection ( 11 , 12 ). In addition, protective immunity to filarial infections in mice is dependent primarily on Th2 responses in mice. Thus, mice lacking IL-4, IL-4R or Stat6 (all deficient in Th2 responses) are all susceptible to infection with Brugia parasites ( 13 , 14 ). Interestingly, IFNγ also appears to play an important role in protection against infection since mice lacking IFNγ, exhibit impairment in the elimination of the parasite ( 13 ). Therefore, protective immunity to filarial infections requires co-ordination of both Th1 and Th2 responses.
One of the most consistent findings in filarial infections is the elevated level of IgE that is observed following L3 exposure ( 15 ). Most of the IgE produced is polyclonal IgE indicating a non- antigen specific induction of IgE producing B cells. ( 16 ). Indeed, these IgE antibodies remain detectable many years after the infection has been treated indicating the presence of long lived memory B cells or plasma cells in filarial infections ( 17 ). IgE production both in mice and humans is absolutely dependent on IL-4 or IL-13. Other isotypes that are commonly elevated in chronically filarial - infected humans are IgG4 and IgG1, the former being most dependent on both IL-4 and IL-10 ( 18 ). The role of B cells in resistance to infection is less clear, although B cells. especially a specialized subset of B cells called B1 B cells, also appear to exert a major role in resistance to infection ( 19 ). Antibodies do play a major role in mediating protection to filarial infections. Thus, in vivo data from mice deficient in IgE, showed increased worm burdens with B. malayi indicating an important role for IgE in host defense ( 20 ). Again using knockout mice models, IgM has also been shown to be crucial for host protection against B. malayi ( 21 ). In the lymphatics and lymph nodes as well as in the circulation, filarial parasites are susceptible to attack by the full range of host innate effector cells, including macrophages, eosinophils and neutrophils. The ability of these cells to kill the parasites is often dependent on one or more isotypes of specific antibody (often IgE but also IgM) and complement. Activated macrophages or granulocytes can release nitric oxide, damaging nitrogen intermediates and reactive oxygen species onto the surface of the parasites, but in vivo killing methods are not yet fully understood.
Dendritic cells are professional antigen-presenting cells that play an essential role in presenting antigen to T cells to initiate immune responses, yet their role in filarial infections is not fully understood. It has been shown that differentiation and maturation of DC in the presence of filarial antigens in vitro can stimulate Th2 responses with downmodulation of IL-12 production ( 22 ). In addition, live parasites have also been shown to induce cell death in human dendritic cells and diminish their capacity to activate CD4 + T cells ( 22 ). Finally, asymptomatic filarial infection is characterized by increased numbers of circulating myeloid dendritic cells (defined as Lineage − , HLA-DR + , CD11c + cells) ( 23 ). On the other hand, human Langerhans’ cells (langerin(+) E-cadherin(+) CD1a(+)) exhibit minimal alterations in the cell surface activation markers or in mRNA expression of inflammation-associated genes, indicating a quiescent initial interaction of the parasite with human epidermal LC ( 24 ).
Macrophages are the other important class of antigen presenting cells that can serve as protective effector cells in bacterial and protozoan infections by their production of nitric oxide and other mediators. A special class of macrophages are known to be induced in filarial infections, characterized by their preferential expression of the enzyme arginase, instead of nitric oxide due to increased activation of arginase-1 by IL-4 and IL-13 ( 25 ). These macrophages, termed alternatively activated macrophages, have a very specific gene expression profile, with the ability to upregulate markers including arginase-1 , chitinase 3-like proteins 3 and 4 (also known as YM1 and YM2 , respectively) and resistin-like molecule-α (RELMα) ( 26 ). These alternatively activated macrophages are known to be important in wound healing and are thought to help limit tissue immunopathology ( 27 ). By virtue of expression of expressing regulatory molecules such as IL-10, TGFβ and programmed cell death 1 ligand 2 (PDL2), these macrophages might play a predominantly regulatory role in filarial infections ( 27 ). Interestingly, these filarial induced macrophages appear to have the ability to expand locally and are less dependent on influx of monocytes from the bloodstream to perform their functions( 28 ). While filarial infection does induce expression of these cells in humans, early interaction of parasites or parasite antigens leads to a predominantly pro-inflammatory response with expression of mainly pro-inflammatory cytokines including TNFα, IL-6 and IL-1β, as well as genes involved in inflammation and adhesion ( 29 ). Studies from murine models of filarial infection and in vitro data indicate that nitric oxide production by macrophages might be a key lethal hit in the host defense against the parasite ( 30 ). Therefore, the induction of alternatively activated macrophages might be an important immune evasion strategy for the parasites. Indeed, monocytes from patients with asymptomatic filarial infection also exhibit hallmarks of alternative activation, with diminished expression of inducible nitric oxide and enhanced expression of arginase-1 , along with increased expression of resistin, mannose receptor C type 1 (MRC-1), macrophage galactose type C lectin (MGL) and chemokine ligand 18 (CCL18). Interestingly, live filarial parasites induce a monocyte phenotype that partly resembles the alternatively activated state seen in infected individuals ( 31 ). Finally, monocytes, especially classical monocytes, appear to be remarkably efficient in antigen-uptake in filarial infections ( 32 ).
Blood eosinophilia is characteristic of filarial infection and is mediated by IL-5 (probably in concert with IL-3 and GM-CSF) ( 33 ). Eosinophils are often the first cell type recruited to the site of infection and eosinophilia occurs characteristically early following infection ( 33 ). Apart from the rapid kinetics of recruitment, eosinophils also exhibit morphological and functional changes attributable to eosinophil activation. These include changes in cell density, increased surface expression of activation markers, enhanced cellular cytotoxicity and release of granular proteins, cytokines, leukotrienes and other mediators of inflammation ( 33 ). Basophils have gained prominence recently due the possible role in Th2 cell differentiation as providers of initial IL-4 and even antigen-presenting cells (APCs). Basophils in humans and mice readily generate large quantities of IL-4, in response to various stimuli, including filarial antigens, with or without dependence on IgE ( 34 ). In murine filarial infections, effector mechanisms involve multiple innate immune cells, with antibodies acting as initiators of immunity by activating Fc receptor expressing cells. Basophils, by their ability to produce high levels of IL-4, act as effectors to promote filarial killing in secondary or challenge infections ( 35 ). Although eosinophils are crucial contributors to the early IL-4 pool, they are also important in protection against primary filarial infections ( 36 , 37 ). The mechanism of protection mediated by eosinophils is thought to antibody-dependent cell mediated cytotoxicity or through release of eosinophil granule contents.
Immune evasion
Filarial parasites exert profound immunoregulatory effects on the host immune system with both parasite-antigen specific and more generalized levels of immune modulation. The main mechanisms of immune evasion include immunosuppression, immunological tolerance and modification of stereotypical Th2 responses ( 3 ). Immunosuppression and immunological tolerance are characterized typically by immunoregulatory cytokine induced suppression of immune responses and a state of immune tolerance in effector T cells, respectively. On the other hand, in the modified Th2 response, antibody isotype switching to the non-inflammatory isotype IgG4 (in humans) and induction of alternatively activated macrophages occurs ( 3 ). It has been shown that patients with lymphatic filariasis have markedly diminished responses to parasite antigens and in addition, some non-specific inhibition of responses to bystander antigens ( 38 ). Thus, while host immunosuppression is usually antigen-specific, chronic infection is often associated with spillover effects on third party antigens as well.
Among the notable immune-evasion strategies, a key one is the secretion of products that modulate host immune function. Phosphorylcholine (PC) is a small hapten-like moiety present in the excretory/secretory products of many helminths and one particular PC containing molecule called ES-62 from filarial worms has been shown to have a wide variety of immunomodulatory properties ( 39 ). Thus, ES-62, can downregulate the proliferation of CD4+ T cells and conventional B cells, decrease IL-4 and IFNγ production, upregulate proliferation and IL-10 production by B1 B cells and condition antigen-presenting cells to drive Th2 differentiation with concomitant inhibition of Th1 responses ( 40 ). Similarly, the presence of cytokine and chemokine like molecules in filarial parasites has been shown to have functional effects on the host innate immune responses as is the case with TGFβ and macrophage migration inhibitory factors (MIF) homologs ( 41 ). In addition, a recent analysis of the filarial parasite genome has identified a remarkable number of human cytokine and chemokine mimics and/or antagonists within the parasite genome. These include members of the interleukin-16 (IL-16) family, an IL-5 receptor antagonist, an interferon regulatory factor, a homolog of suppressor of cytokine signaling 7 (SOCS7) and two members of the chemokine-like family. Moreover, other potential immunomodulators have been demonstrated to be present in the filarial genome, including serpins and cystatins (modulation of antigen processing and presentation to T cells), indoleamine 2,3-dioxygenase (IDO) genes (potent immunomodulatory molecule), and Wnt family of developmental regulators (modulation of immune activation)( 42 ).
The induction of regulatory T cells, modulation of effector T cell and antigen-presenting cells and apoptosis of responder cells ( 43 ) have been suggested to be the major factors influencing the regulation of the immune response in filarial infection. Regulatory T cells (both natural and adaptive) have been postulated to play a role in the immune evasion mechanism of the parasite. IL-10 and TGFβ, both factors associated with regulatory T cells, are elicited in response to helminth infections, and in vitro neutralization of IL-10 and TGFβ has been demonstrated to partially restore both T cell proliferation and cytokine production in lymphatic filariasis ( 44 ). Similarly, evidence from mouse models argues for a major role of natural Tregs (CD25 + Foxp3 + Treg cells) in immunity during filarial infections ( 45 - 47 ). In murine filarial infections, parasite survival is directly linked to Treg activity and immunity to infection can be restored by elimination of Tregs ( 45 ). Effector T cell responses can be turned off or modulated through a variety of mechanisms including through CTLA-4 and PD-1. Interestingly, increased expression of CTLA-4 and PD-1 has been demonstrated in human filarial infections, and blocking of CTLA-4 can restore partially a degree of immunological responsiveness in cells from infected individuals ( 48 , 49 ). Similar findings have also been observed in murine models of filarial infection ( 50 ). Finally, classical signs of anergy has been demonstrated in T cells of filarial infected individuals with decreased IL-2 production and increased expression of E3 ubiquitin ligases ( 49 ).
Filarial parasites induce downmodulation of MHC class I and class II as well as cytokines and other genes involved in antigen-presentation in dendritic cells, thereby rendering them less capable of activating CD4+ T cells ( 22 ). In addition, live parasites have also been shown to cause downregulation of MHC class II and IL-8, I and multiple genes involved in antigen presentation in skin-resident LC ( 51 ). Filarial parasite interaction with macrophages has been shown to induce alternatively activated macrophages. By virtue of expression of expressing regulatory molecules such as IL-10, TGFβ and programmed cell death 1 ligand 2 (PDL2), these macrophages may also have a predominantly regulatory role in filarial infections ( 52 ). These anti-inflammatory macrophages can suppress T cell responses through arginase-1 production and PDL2 expression and inhibit classical macrophage inflammation and recruitment through arginase-1, RELMα, triggering receptor expressed on myeloid cells 2 (TREM2) and other molecules ( 53 ). Another mechanism of immune evasion is the ability of filarial parasites to induce host cell apoptosis ( 54 ). Apoptosis of CD4+ T cells has been demonstrated in vivo in experimental models of filarial infection in mice. In addition, Brugia microfilariae have been shown to interact with dendritic cells and NK cells and subsequently induce their apoptosis ( 22 , 55 ).
One of the main characteristics of asymptomatic or subclinical filarial infection is the modulation of TLR expression and function in a variety of cell types including B cells, T cells and monocytes ( 56 , 57 ). Either homeostatic or antigen-stimulated expression of certain TLRs was shown to be diminished in B cells, T cells and monocytes of filarial-infected individuals. In addition, TLR stimulation of both APCs and T cells leads to diminished activation / cytokine production, indicating a state of immune regulation. Furthermore, live filarial parasites have the capacity to downregulate TLR expression (specifically TLR3 and 4) on dendritic cells as well ( 58 ). This is accompanied by an impaired ability of dendritic cells to produce certain cytokines in response to TLR3 and 4 ligands. The diminished expression and function of TLR on immune cells is thought to be a likely consequence of chronic antigen stimulation and probably serves as a novel mechanism to protect against the development of pathology in filarial disease ( 59 ).
While most of the immunological studies in filarial infections have focused on filarial antigen induced immune responses, the study of the immune responses engendered by live parasites provides some interesting details ( 49 ). Live parasites cause a significant impairment of both Th1 and Th2 cytokines in response to both L3 and Mf stages with diminished production of Th1 (IFNγ and TNF-α) and Th2 ( IL-4 and IL-5) cytokines. This is accompanied by an impaired induction of T-bet (the master Th1 transcription factor) and GATA-3 (the master Th2 transcription factor) mRNA and by significantly increased expression of Foxp3, TGFβ, CTLA-4, PD-1, ICOS and IDO. In addition, the compromise of effector T cell function is mediated by the enhanced induction of anergy-inducing factors – cbl-b, c-cbl, Itch and Nedd4. Finally, blocking CTLA-4 or neutralizing TGFβ restored the ability to mount Th1/Th2 responses and reversed the induction of anergy-inducing factors. Thus, a variety of regulatory factors including IL-10, TGFβ, nTregs (perhaps via PD-1 and CTLA-4) have been implicated in the downmodulation of immune responses in patent filarial infection and might have a potentially vital role in the establishment of chronic, asymptomatic infection.
Pathogenesis of filarial disease
The most severe clinical manifestations of lymphatic filariasis are lymphedema and elephantiasis. The first major insights into the role of lymphatic damage in the pathogenesis of lymphatic filarial disease came from studies using Brugian infections of animals. Infection of normal or nude (lacking T cells) mice resulted in lymphangitis and peri-lymphangitis in both groups of mice with acute and chronic inflammation predominating in the former ( 60 ). Interestingly, since normal mice are not permissive to infection, lymphangiectasia was observed to progress only in nude mice. While infection of nude mice was characterized predominantly by lymphangiectasia, reconstitution of these mice with spleen cells from normal mice (thereby restoring normal adaptive immunity) resulted in progressive fibrosis, obliterative lymph thrombus formation, interstitial infiltrates and extensive perilympangitis ( 61 ). Similarly, studies using SCID mice (lacking both T and B cells) showed that lymphangitis and lymphangiectasia were classical features of infection in the absence of adaptive immunity, and that reconstitution with spleen cells from normal mice resulted in progressive disease ( 9 ).
Pro-inflammatory cytokines of innate origin also appear to play an important role in brugian infection since infection of nude mice results in elevated levels of IL-1, IL-6, TNF-α and GM-CSF in lymph fluid ( 62 ). Therefore, innate cytokines appear to play a prominent role in the initiation of pathology in filarial-infected animal models. The importance of pro-inflammatory cytokines, possibly of innate origin, in the pathogenesis of lymphedema, has been further strengthened by a series of studies in humans in either the early or late stages or lymphedema. Studies have shown that individuals with chronic lymphatic pathology have elevated levels of C-reactive protein (an acute phase protein, indicating an acute inflammatory response), pro-inflammatory cytokines such as TNF-α, IL-6 and soluble TNF receptor, endothelin-1, IL-2, as well as IL-8, MIP-1α, MIP-1β, MCP-1, TARC and IP-10 in the peripheral circulation ( 6 ). Similarly, while patients with both acute and chronic manifestations of LF have elevated circulating levels of IL-6 and IL-8, only those with chronic disease manifestations have elevated levels of sTNF receptors ( 63 ). Another important mechanism of immune activation in chronic infections is the occurrence of microbial translocation with elevations in the circulating levels of microbial products. Microbial translocation across the intestine or across the lymphatics could possibly contribute to inflammation and innate immune activation. Indeed, we have shown that increased circulating levels of LPS (which serves as a marker for microbial translocation) and decreased levels of LPS-binding protein (LBP) are characteristic features of filarial lymphatic pathology ( 64 ). We have also demonstrated that this process is associated with development of an acute-phase response and the presence of markers of inflammation in plasma – CRP, alpha-2 macroglobulin, serum amyloid protein-A and haptoglobin ( 64 ). Moreover, increased serum levels of proinflammatory cytokines – IL-1β, IL-12, TNF-α and IL-6 are associated with progressive immune activation in filarial pathology. Since filarial lymphedema is known to be associated with increased bacterial and fungal loads in the lymphatics, we postulate that microbial translocation across the damaged lymphatics in filarial lymphedema is a novel source of immune activation.
Apart from systemic immune activation, progressive fibrosis and extracellular matrix remodeling is another salient feature of filarial pathology. Matrix metalloproteinases (MMPs) are proteolytic enzymes that control matrix remodeling and collagen turnover. These MMPs and their inhibitors (tissue inhibitors of metalloproteinases [TIMPs]) are produced by a variety of cell types including macrophages, granulocytes, epidermal cells, and fibroblasts. The dysregulation of MMPs and TIMPs is known to underlie the development of pathology in several infections, including viral, bacterial, spirochetal, protozoan, fungal, and parasitic infections. Along the same lines, recent data suggests that an increase in circulating levels of MMPs and TIMPs is characteristic of the filarial disease process and that that altered ratios of MMP/TIMP are an important underlying factor in the pathogenesis of tissue fibrosis in filarial lymphatic disease. In addition, this is correlated with elevated levels of Type 2 cytokines known to be intimately involved in fibrosis – IL-5, IL-13 and TGFβ ( 65 ). Another study has also examined the alterations in pro-fibrotic factors in filarial pathology and revealed that increased levels of basic fibroblast growth factor (bFGF) and placental growth factor (PIGF) can also occur in filarial lymphedema patients ( 66 ). Thus, filarial pathology arises out of a complex early interplay between the parasite and the host's innate responses and its tissue homeostasis.
Studies in animals also implicate an important role for endothelial cells in pathogenesis of lymphatic dysfunction since these cells exhibit morphological alterations upon chronic infection. Indeed, live filarial parasites (and their excretory/ secretory products) induce activation, proliferation and tube formation in lymphatic endothelial cells ( 67 ). Moreover, only serum from patently infected or diseased individuals was shown to induce significant lymphatic endothelial cell (LEC) proliferation. This lymphatic remodeling recapitulates the observations seen in vivo in immunodeficient mice. Vascular endothelial growth factor (VEGF) family members have also been implicated in lymphangiogenesis. It was recently shown that lymphaticendothelial specific VEGF-C levels are significantly elevated in individuals with filarial disease ( 68 ). The other VEGF family member that has been implicated to play a role in filarial disease is VEGF-A ( 69 ). Elevated levels of VEGF-A and endothelin-1 have been observed in the serum of filarial-infected individuals and more specifically, VEGF-A has been implicated to play a role in the development of hydrocele due to its ability to induce increased vascular density, enhance leucocyte adhesion and promote lymphangiogenesis ( 70 ). Thus, excess secretion of pro-inflammatory cytokines and angiogenic factors like VEGF-A could result in extravasation and accumulation of fluids, plasma, and lymph from the blood and lymphatic vessels into the scrotum resulting in the formation of hydrocele. Other angiogenic factors such as angiopoietins-1 and 2 are also found at elevated levels in individuals with filarial-induced pathology ( 66 ).
In terms of cellular subsets, it was first discovered that individuals with chronic lymphedema have increased frequencies of activated CD8 + T cells (expressing HLA-DR) in the peripheral blood ( 71 ). Later, it was also shown that the frequency of CD8 + T cells in tissues (including skin and subcutaneous tissues) was increased as well ( 72 ). Indeed, biopsy specimens from affected tissues exhibited increased levels of VCAM-1, and PBMC supernatants from diseased individuals showed the capacity to up-regulate both MHC-Class I molecules and VCAM-1 on endothelial cell cultures ( 73 , 74 ). Moreover, TCR Vβ phenotyping revealed a biased TCR repertoire in the T cells infiltrating the affected tissues in diseased individuals ( 75 ). In addition, examination of chemokine receptor expression on T, B and NK cells revealed a significant increase in the frequencies of circulating CCR9 - expressing T and B cells ( 76 ). Since, CCR9 is not normally expressed on circulating T and B cells. these results suggest that chemokine receptors (particularly CCR9) are involved in the pathogenesis of lymphatic filarial disease and that trafficking of particular cellular subsets may influence clinical outcome. Unpublished data utilizing mutliparameter flow cytometry has failed to reveal any significant difference in the frequencies of circulating naïve, effector memory and central memory CD4 + and CD8 + T cells in patients with filarial disease compared to those with longstanding infection. Thus, alterations in T cell numbers and function, especially at the site of pathology, are probably of major importance in pathogenesis.
Using multi-color flow cytometry we have been able to show that the frequency of Th1 cells (CD4 + T cells expressing either IFNγ or IL-2 or TNF-α); Th9 cells (CD4+ T cells expressing IL-9 and IL-10); Th17 cells (CD4+ T cells expressing IL-17) and Th2 cells (CD4+ T cells expressing IL-22) is significantly enhanced in filarial pathology. This is accompanied by a concomitant decrease in the frequency of Th2 cells (CD4 + T cells expressing IL-4 or IL-5 or IL-13) both at homeostasis and following parasite antigen stimulation (data not published). Although less well studied than Th1 cells, Th17 cells might also have an important role in the pathogenesis of disease in filarial infection since PBMC from individuals with pathology (but not asymptomatic patients) express significantly higher levels of the Th17 associated cytokines as well as the master transcription factor - RORC at the mRNA level ( 77 ). Finally, pathology in lymphatic filariasis is also associated with expanded frequencies of Th9 cells, CD4 + T cells that express both IL-9 and IL-10 but not IL-4 and this frequency exhibits a positive correlation with the severity of lymphedema in filarial infections (unpublished results). Therefore, immunopathology in lymphatic filariasis appears to be mostly associated with poor regulation of effector CD4+ and CD8+ T cells that can unleash pro-inflammatory Th1, Th9 and Th17 type immune responses. How, these pro-inflammatory Th1, Th9 and Th17 cells interact with innate cells, endothelial cells and other target cells to initiate and propagate lymphatic damage and tissue fibrosis remains to be elucidated.
Conclusions
Filarial infections are a classic example of chronic infections in which complete elimination of all parasites is rarely achieved, presumably since sterilizing immunity might necessitate deleterious host immune responses. Therefore, immune-mediated pathology is often associated with disease manifestations in these infections. The optimal host response is one which balances parasite control at the levels in which the parasite load can be tolerated and maintenance of immune homeostasis without irreparable tissue damage. Filarial infections, for the majority of those infected, reflect immune system-parasite balance, a balance that, when it fails, results in significant immune-mediated inflammation and pathology. Interestingly, this is also reflected in the disease manifestations of the related filarial parasite, Onchocerca volvulus, where chronic, asymptomatic infection is associated with loss of parasite control and the hyper-reactive, pro-inflammatory state is associated with severe chronic papular dermatitis and hyperpigmenation (sowda) ( 78 ).

Regulation of the immune responses in filarial infections. The complex outcome of the interaction between the filarial parasite and the host immune system determines the immunological outcomes including: (a) protection against infection; (b) parasite specific T cell hypo-responsiveness and alteration of APC function; (c) chronic infection; (d) protection against pathology and (e) anti-inflammatory bystander suppression.
Acknowledgements
This work was supported by the Intramural Research Program of the Division of Intramural Research, National Institute of Allergy and Infectious Diseases, National Institutes of Health.
Conflict of Interest Disclosure
Because S. Babu and T. B. Nutman are government employees and this is a government work, the work is in the public domain in the United States. Notwithstanding any other agreements, the NIH reserves the right to provide the work to PubMedCentral for display and use by the public, and PubMedCentral may tag or modify the work consistent with its customary practices. You can establish rights outside of the U.S. subject to a government use license.

IMAGES
VIDEO
COMMENTS
1. Introduction. Host immune responses to parasitic infections are complex. Parasites include protozoa, helminths, and insects. Previously, the author proposed a framework for all the known host immunological pathways and their roles in the immune responses against four specific types of pathogens and the corresponding four specific types of hypersensitivities [1,2].
Unlike bacterial infection, the immune responses against parasitic infection are mostly nonprotective immune responses, which may lead to severe harmful effect in the host, and it may last for a very long course of time. Both intracellular and extracellular parasites have evolved themselves to cope up against the complex host immune response ...
Metazoan parasites typically induce a type 2 immune response, characterized by T helper 2 (Th2) cells that produce the cytokines IL-4, IL-5 and IL-13 among others. The type 2 response is host protective, reducing the number of parasites either through direct killing in the tissues, or expulsion from the intestine.
Infections caused by parasitic pathogens are a global health problem that affects more than a quarter of the world's population (), yet effective antiparasitic therapeutics are limited and often come with severe adverse reactions.Similarly, vaccines are limited for any food or water-borne parasitic infection ().Immune responses initiated by the innate immune system in the intestinal tissue ...
In many instances, the ability to successfully combat parasitic infections requires that the host mount an effective inflammatory response against the parasite, while limiting potential tissue damage to the host. Acquisition of infection, clinical severity and outcome of a parasitic disease often depends on innate and acquired host immunity.
Disease tolerance is an evolutionarily conserved defence strategy against infection that does not exert a direct negative effect on the host pathogen load. Disease tolerance relies on tissue ...
Parasitic diseases continue to be a major cause of morbidity and mortality worldwide, with billions of people at risk of infection, often with multiple parasites (https://www.who.int). Despite this global impact, there are limited treatment options for most infections and only one human vaccine, which is moderately effective against malaria (Stutzer et al., 2018;Zavala, 2022). The key to ...
The TH1 response is important in protection against pre-erythrocytic stage of Plasmodium apart from protection against Leishmania and Toxoplasma infections. 2. ... Marichal T, Galli SJ. IgE and mast cells in host defense against parasites and venoms. Semin Immunopathol. 2016;38:581-603. Article CAS PubMed PubMed Central Google ...
Introduction. The immune system is an essential defence against infection, but it is a double-edged sword. Inappropriate or over-exuberant immune responses can be as harmful as the infections they are meant to protect against, and the immune system includes a rigorous set of regulatory mechanisms that temper its activity 1-3.Parasite infections present a complex challenge to the immune system ...
Abstract. An organism's physiological equilibrium is critically reliant on its immune system, which provides protection against parasites, pathogens, toxic substances, and cancerous cells and allows recovery from injuries. The immune response is, however, not cost free. It demands various kinds of resources, but also more subtle costs.
As previously mentioned, a complex interaction occurs between the different stages of the parasite (Leishmania) and the antigen-presenting cells (APCs) of the host, which is important for determination of the clinical outcome of the disease.The immune response to Leishmania mainly depends on a type I immune response that is characterized by the initial production of interleukin-12(IL-12) by ...
Today, despite available treatments, more than 2 billion people are suffering from chronic infections with parasites, resulting in considerable morbidity [].Chronic infections develop because these parasites escape destruction by the immune system, arguably helped by their complex biology [].Parasitic infections and the host's immune responses are the result of dynamic co-evolution of the ...
The parasite host response is the process by which a host interacts with and responds to parasites that it encounters. It includes various mechanisms, including immune mechanisms that are elicited ...
Parasites have evolved a wide range of mechanisms that they use to evade or manipulate the host's immune response and establish infection. The majority of the in vivo studies that have investigated these host-parasite interactions have been undertaken in experimental animals, especially rodents, which were housed and maintained to a high microbiological status.
Abstract. Parasitic protozoans such as Trypanosoma and Plasmodium cause many of the most prevalent and dreadful diseases in humans. The survival of these parasitic protozoans relies on the successful evasion of the host organism immune system. These include invasion and replication with host cells, as well as alterations of their surface proteins.
of no consequence to either host or parasite, or (4) definitively harmful to the host and resulting in pathology and disease manifestations. In this paper, the subject of immune response to parasites is briefly reviewed, with selected examples of the extreme variety of effector mechanisms that the host employs against parasites. Effector Mechanisms
Combating Parasites: Immune Response and Inflammation Abstract An organism's physiological equilibrium is critically reliant on its immune system, which provides protection against parasites, pathogens, toxic substances, and cancerous cells and allows recovery from injuries. The immune response is, however, not cost free.
Many successful parasites exhibit antigenic variation to avoid immune elimination during infection. The most well-known example of immune evasion by parasites is antigenic variation by the African trypanosome, which for nearly a century has provided the classical paradigm for microbial antigenic variation as a means of escaping host immunity.
The parasite's development and progression through the life cycle is adapted to the physiology and behaviour of the host, and the higher the parasite load the greater the severity of disease in the host. Parasites must reach new hosts and have evolved a variety of strategies to achieve this. For some there is a direct life cycle, with the parasite passing from one member of the host species to ...
Studies of the immune response against helminths are of great interest in understanding interactions between the host immune system and parasites. Effector immune mechanisms against tissue-dwelling helminths and helminths localized in the lumen of organs, and their regulation, are reviewed. Helminth infections are characterized by an ...
However, with repeated infection, the host's immune responses are dampened: helminths can effectively block alarmin production and TLR function, ultimately preventing the T helper (Th) type 2 immune response. Allergy, Autoimmunity, and Allograft Rejection. Helminth-induced immunomodulation can be beneficial for the host, as well as the parasite.
The canonical host immune response to filarial parasites in both mice and humans is of the T helper 2 (Th2) type and involves the production of cytokines - IL-4, IL-5, IL-9, IL-10 and IL-13, ... IgM has also been shown to be crucial for host protection against B. malayi . In the lymphatics and lymph nodes as well as in the circulation ...
In some cases, products from parasitic protozoans are responsible for immunosuppression observed in the host organism and it is associated with antigenic mimicry, which is usually linked to diseases induced by these parasites. Other immune responses to protozoans includes activation of T cells and inflammatory cascade of host organism.