Advertisement

- Previous Article
- Next Article

Introduction
The largest cells of the body, the textbook model for muscle size regulation, the controversies, a new model for muscle size regulation, muscle memory, implications for sport and public health, future perspectives, muscle memory and a new cellular model for muscle atrophy and hypertrophy.
Competing interests
The author declares no competing or financial interests.
- Split-screen
- Article contents
- Figures & tables
- Supplementary Data
- Peer Review
- Open the PDF for in another window
- Get Permissions
- Cite Icon Cite
- Search Site
Kristian Gundersen , Stan L. Lindstedt , Hans H. Hoppeler; Muscle memory and a new cellular model for muscle atrophy and hypertrophy. J Exp Biol 1 January 2016; 219 (2): 235–242. doi: https://doi.org/10.1242/jeb.124495
Download citation file:
- Ris (Zotero)
- Reference Manager
Memory is a process in which information is encoded, stored, and retrieved. For vertebrates, the modern view has been that it occurs only in the brain. This review describes a cellular memory in skeletal muscle in which hypertrophy is ‘remembered’ such that a fibre that has previously been large, but subsequently lost its mass, can regain mass faster than naive fibres. A new cell biological model based on the literature, with the most reliable methods for identifying myonuclei, can explain this phenomenon. According to this model, previously untrained fibres recruit myonuclei from activated satellite cells before hypertrophic growth. Even if subsequently subjected to grave atrophy, the higher number of myonuclei is retained, and the myonuclei seem to be protected against the elevated apoptotic activity observed in atrophying muscle tissue. Fibres that have acquired a higher number of myonuclei grow faster when subjected to overload exercise, thus the nuclei represent a functionally important ‘memory’ of previous strength. This memory might be very long lasting in humans, as myonuclei are stable for at least 15 years and might even be permanent. However, myonuclei are harder to recruit in the elderly, and if the long-lasting muscle memory also exists in humans, one should consider early strength training as a public health advice. In addition, myonuclei are recruited during steroid use and encode a muscle memory, at least in rodents. Thus, extending the exclusion time for doping offenders should be considered.
A memory process entails encoding, storing and retrieving information. For vertebrates, the modern view has been that this process only occurs in the brain, where it is probably related to long-lasting changes in synaptic efficiency. There has been a notion that other organs or the body as a whole might also have a form of ‘body memory’. For example, in popular culture it has been envisioned that transplanted organs might confer some form of personality transfer.
The concept has also been used in more serious psychological literature on child abuse, where the bodies of the victims supposedly remember the traumatic incidence ( Koch et al., 2012 ). It has been unclear whether the term is used literally or metaphorically, but in any case, the notion has been largely discredited and body memory has been considered to be a pseudoscientific concept ( Smith, 1993 ).
More credible is the use of the term memory in the immune system to describe the fact that on the second encounter of an antigen, the immune response is stronger and more rapid. This memory is stored in memory immune cells; for example, memory T cells exposed to a specific antigen have a different molecular makeup compared with naive T cells ( Mackay, 1999 ).
The term cell memory has also been used to describe irreversible programming of cells such as stem cells, and is attributed to epigenetic mechanisms such as histone and DNA modifications other than changes in base sequence (e.g. Alvarez and Margulies, 2014 ; Li and Zhang, 2014 ). For example, it has been demonstrated that fibroblasts in culture maintain a specific gene expression pattern depending on where in the body they are taken from, and this has been dubbed a positional memory ( Chang et al., 2002 ). The more traditional term for such phenomena is cell differentiation. Although some forms of cell differentiation might fill the formal criteria for memory as defined in the first paragraph above, perhaps the term should be reserved for latent, lasting, adaptive changes that are caused by environmental factors.
In relation to skeletal muscle, René Descartes (1596-1650) wrote: ‘The lute players have part of their memory in their hands, because the facility to move and bend their fingers in various ways which they have acquired by habit, helps them to remember passages that require them to move their fingers in a way in order to play them’ ( Koch et al., 2012 ). In this statement, Descartes seems to believe that there is a memory in the fingers themselves, and such ideas have probably led to the term muscle memory. Used this way, it is an unfortunate term, because our current understanding is that learning to play the lute and similar tasks is a form of motor learning in the central nervous system (CNS), and not the muscles. Thus, what has scientifically been called muscle memory so far is really synonymous to motor learning. Motor learning has been treated in a recent review ( Diedrichsen and Kornysheva, 2015 ) and is outside the scope of this paper.
The term muscle memory has also been used for the ability to rebuild muscle mass and strength, as previous strength exercise seems to make it easier to regain muscle mass later in life even after long intervening periods of inactivity and mass loss ( Staron et al., 1991 ; Taaffe and Marcus, 1997 ). This phenomenon has hereto, however, also been solely attributed to motor learning in the CNS ( Rutherford and Jones, 1986 ). There is clearly a CNS component to the muscle memory of strength training, as the increase in force may precede the increase in muscle mass, possibly by altering spinal motor neuron excitability, and induce synaptogenesis within the spinal cord ( Adkins et al., 2006 ). For strength, however, the build-up of muscle protein is crucial, and a purely neural mechanism has not seemed satisfactory.
Results from recent experiments suggest that there is a form of cellular memory in the muscle cells themselves explaining the phenomenon that muscle mass previously obtained is easily regained, and that the cellular mechanism for this ‘memory’ is related to the number of myonuclei ( Bruusgaard et al., 2010 ; Egner et al., 2013 ; Gundersen, 2011 ).
Muscle cells are peculiar in being by far the largest cells of the mammalian body. In mice, limb muscle fibres have a typical volume of 5 nl ( Utvik et al., 1999 ). In larger mammals, volumes can be much higher; e.g. a cylinder with a diameter of 50 µm and a length of 0.5 m has a volume of ∼10 3 nl ( Bruusgaard et al., 2003 ). Most other mammalian cells are more spherical, with diameters in the range of 5–20 µm and volumes ranging from 10 −5 to 10 −3 nl, i.e. many orders of magnitude smaller than a muscle fibre. The muscle cells have multiple nuclei, however, and constitute one of the few syncytia in the mammalian body.
It has been suggested since the 19th century that a nucleus can serve only a certain volume of cytoplasm (e.g. Strassburger, 1893 ), and it was recently argued that the link between DNA content and cell volume is a fundamental principle in biology ( Gregory, 2001 ). In muscle it is believed that each nucleus serves a certain domain, and it has been shown that some proteins are localized to the site of expression both in vitro ( Hall and Ralston, 1989 ; Pavlath et al., 1989 ; Ralston and Hall, 1992 ) and in vivo ( Gundersen et al., 1993 ; Merlie and Sanes, 1985 ; Sanes et al., 1991 ). Each nucleus is surrounded by a synthetic machinery that seems to remain localized ( Pavlath et al., 1989 ).
In its strictest version, this nuclear domain theory has implied that a nucleus supports a constant volume of cytoplasm. We have studied the naturally occurring range of fibre sizes of limb muscles of the mouse using in vivo imaging. In the slow/oxidative soleus muscle of young adult animals (2 months), the strict nuclear domain theory was largely confirmed as the number of nuclei was reasonably proportional to the cytoplasmic volume, but in the fast/glycolytic extensor digitorum longus, the number of nuclei varied proportional to fibre surface, and thus large fibres had systematically larger cytoplasmic volume domains than smaller fibres ( Bruusgaard et al., 2003 ). Similarly, various degrees of correlation between fibre size and number of myonuclei have been found in fixed and isolated fibres from young animals ( Brack et al., 2005 ; Mantilla et al., 2008 ; Wada et al., 2003 ).
Notably, the common observation that there is at least some correlation between size and number of nuclei is not universal. Thus, in older mice (14–18 months) there was no correlation between fibre size and the number of nuclei, in spite of a fourfold variability in size ( Bruusgaard et al., 2006 ; Wada et al., 2003 ). We speculate that the number of nuclei displayed in these older mice reflects not only the acute size of the fibre, but also its history, as a form of memory.
The syncytial nature of muscle fibres might be related to the lack of a long-distance transport system for proteins within these large cells. Thus, the myonuclei are optimally positioned as to minimize transport distances within each fibre ( Bruusgaard et al., 2003 , , 2006 ), and perturbation in the positioning of nuclei leads to impaired muscle function ( Metzger et al., 2012 ).
The syncytial nature and the correlation between nucleic number and cell volume, observed at least under some circumstances, suggest that there is a rate-limiting step related to each nucleus’ capacity for protein synthesis. In particular, during hypertrophic growth it does not seem plausible that, for example, one nucleus could provide all the RNA required to support the vast cytoplasmic volume of muscle fibres, but the nature of the bottleneck is not known.
Interestingly, it was recently suggested that ribosome biogenesis plays a central role during muscle hypertrophy ( Chaillou et al., 2014 ). For example, the 47S pre rRNA transcribed by Pol I is increased during overload hypertrophy ( von Walden et al., 2012 ). rRNA accounts for 70% of all transcription, and most steps in the assembly of the ribosomal subunits take place in the nucleolus, where rRNAs are transcribed as large precursors, which undergo extensive nucleotide modification. In addition, more than 200 non-ribosomal, resident nucleolar proteins are required to process and modify the rRNAs and to aid their assembly with the ∼80 ribosomal proteins ( Granneman and Tollervey, 2007 ). These processes require efficient trafficking across the nuclear membrane. In growing yeast, each nuclear pore is believed to import 1000 ribosomal protein molecules per minute, and export ∼25 ribosomal subunits per minute ( Warner, 1999 ). Although the numbers are probably less dramatic in mammalian cells, such high-throughput processes in the nucleus provide many good candidates for steps that might be limiting hypertrophic growth and act as a bottleneck if the number of nuclei is not sufficiently high.
Changes in muscle fibre size are accomplished by altering the balance between protein synthesis and proteolysis ( Fig. 1 ). The molecular signalling mechanisms regulating these processes have been the subject of several recent reviews (e.g. Cohen et al., 2015 ; Egerman and Glass, 2014 ; Gundersen, 2011 ; Schiaffino et al., 2013 ), and the focus has mainly been on regulating protein degradation and synthesis per nucleus.

Major determinants of muscle fibre size. Changes in fibre size occur by changing the balance between protein synthesis and protein degradation. Total protein synthesis is, by definition, the product of the number of myonuclei and synthesis per nucleus.
A corollary to the idea of constant myonuclear domains is that the number of nuclei should change in proportion to size during atrophy and hypertrophy. This is reflected in the current textbook model for the regulation of muscle mass, which is illustrated in Fig. 2 . The model implies that during hypertrophic growth, myonuclei are recruited from muscle stem cells residing inside the fibre basal lamina (hence called satellite cells), resulting in a large fibre with many myonuclei. The higher number of nuclei should contribute to the increase in protein synthesis because total protein synthesis is the product of synthesis per nucleus and the number of nuclei ( Fig. 1 ).

Current textbook model for the cell biology of hypertrophy and atrophy. According to the model, when fibres grow as a response to a hypertrophic stimulus, myonuclei are recruited from satellite cells to support the larger cytoplasmic volume. During atrophy, the ‘excess’ nuclei are removed from the syncytium by selective nuclear apoptosis. In its strictest interpretation, the number of myonuclei are regulated such that the myonuclear domain volume is the same for the fibre in its atrophic and hypertrophic states. Note that the model is completely reversible; there is no apparent hysteresis or memory.
During atrophy, the model suggests that myonuclei are eliminated by apoptosis ( Fig. 2 ). This would have to be a selective nuclear apoptosis of some of the nuclei within the intact muscle fibre. Notably, according to this model, a fibre undergoing hypertrophic growth, e.g. during strength exercise, and then returning to initial size will appear identical to a fibre that had never undergone growth. It ends up where it started, as a small fibre with few nuclei. There is no apparent hysteresis or memory according to this model. However, both the ascending and the descending limbs of the model have now become controversial.
Is recruitment of myonuclei obligatory for hypertrophy?
It seems generally accepted that myonuclei are added under many hypertrophic conditions ( Allen et al., 1995 , , 1999 ; Bruusgaard et al., 2010 ; Cabric et al., 1987 ; Cabric and James, 1983 ; Cheek et al., 1971 ; Enesco and Puddy, 1964 ; Giddings and Gonyea, 1992 ; Kadi et al., 1999 ; Lipton and Schultz, 1979 ; McCall et al., 1998 ; Moss, 1968 ; Moss and Leblond, 1970 ; Roy et al., 1999 ; Schiaffino et al., 1976 ; Seiden, 1976 ; Winchester and Gonyea, 1992 ). However, the growth differs from the model shown in Fig. 2 in that the increase in number of myonuclei precedes the radial growth rather than lagging it, as has been demonstrated both with 3 H-thymidine labelling ( Aloisi et al., 1973 ) and in vivo imaging ( Bruusgaard et al., 2010 ). Thus, the myonuclear domain is temporarily decreased during the growth phase.
Although this time course might suggest that the increased number of myonuclei is causally related to the build-up of muscle mass, it has been debated whether the addition of myonuclei is obligatory for hypertrophic growth. According to literature from the early 1990s and onwards, hypertrophy is abolished or attenuated when satellite cells are ablated by X-ray irradiation ( Adams et al., 2002 ; Barton-Davis et al., 1999 ; Phelan and Gonyea, 1997 ; Rosenblatt and Parry, 1992 ; Rosenblatt et al., 1994 ; but see also Lowe and Alway, 1999 ; Rosenblatt and Parry, 1993 ). However, this approach was criticized, as it was suggested that the irradiation might have affected other growth mechanisms in addition to ablating satellite cells.
After a comprehensive debate in the Journal of Applied Physiology in 2007 ( Bodine, 2007 ; Hikida, 2007 ), it was concluded that limitations in current methodology made it difficult to reach final conclusions on whether an increase in the number of myonuclei is required for hypertrophy ( O'Connor et al., 2007 ).
More recently, the debate was rejuvenated by several transgenic models displaying large fibres without a corresponding increase in the number of myonuclei, such as mice overexpressing the proteins Ski ( Bruusgaard et al., 2005 ), Akt ( Blaauw et al., 2009 ) or junB ( Raffaello et al., 2010 ), as well as myostatin-null mice ( Amthor et al., 2009 ). However, for Ski and myostatin the hypertrophy is not fully functional, as the specific force is reduced ( Amthor et al., 2007 ; Charge et al., 2002 ; Mendias et al., 2011 ). Although it was not measured, the same is probably true for junB, as it might also act by inhibiting myostatin ( Raffaello et al., 2010 ).
Akt mice seemed to have normal specific force after 3 weeks of overexpression ( Blaauw et al., 2009 ), but it is unclear whether this condition is sustainable over longer periods ( Blaauw and Reggiani, 2014 ). Similarly, specific force appeared normal after 2 weeks of overload hypertrophy in mice where 90% of satellite cells were ablated using an inducible Pax7–diphtheria toxin transgene ( McCarthy et al., 2011 ). However, the latter study is problematic because 30% of the fibres in the control material were regenerating, questioning the validity of the observations for pre-existing fibres. Also, I have calculated the average hypertrophy both in the control group and the group without satellite cells to be only 10% in the study, so the paper has little relevance to hypertrophy in excess of that. Even though the authors more recently published a long-term study ( Fry et al., 2014 ), the interpretations for hypertrophy in pre-existing fibres are confounded by the high incidence of regenerative growth in the model used.
In conclusion, myonuclei are recruited before growth under many hypertrophic conditions, but it is still unclear whether one can also have a functional and sustainable hypertrophy without recruiting new myonuclei. As discussed in the next paragraph, nuclei are not lost during detraining or other atrophy conditions (the descending part of the model shown in Fig. 2 ). This is the core of the mechanism for muscle memory, and it allows for hypertrophic growth without recruitment of myonuclei during re-training, not because more nuclei are not needed in large fibres (as discussed above), but because the nuclei are already there.
Are myonuclei lost during atrophy?
Most of the focus on atrophy and atrophy prevention has been on satellite cells and their activation, i.e. multiplication and differentiation into myoblasts. Based on the model shown in Fig. 2 , atrophy was interpreted as a degenerative disease where myonuclei need to be replenished, for example, by stem cell treatment. Some authors have even blurred the conceptual distinction between myonuclei proper and satellite cells, and are talking about muscle nuclei. This is unfortunate, because the functionally important nuclei for synthesis of muscle proteins are the myonuclei, not the satellite cells. Thus, the ability to distinguish the myonuclei both conceptually and physically becomes crucial. Precise identification of myonuclei is not trivial because at least half of the nuclei of muscle tissue are in other cell types. The descending limb of the model in Fig. 2 has become highly controversial, and previous misconception is likely to be largely due to difficulties in identifying myonuclei.
Using in vivo time-lapse imaging where myonuclei in specific segments of the same muscle fibre could be followed over time ( Bruusgaard and Gundersen, 2008 ), we observed that the number of myonuclei remained constant when fibres were followed for up to 28 days after denervation, resulting in a volume loss of more than 50%. One example is shown in Fig. 3 . Similar observations were made for both fast and slow muscle, and in several different atrophy models such as nerve impulse block with tetrodotoxin, antagonist ablation ( Bruusgaard and Gundersen, 2008 ), hind limb suspension ( Bruusgaard and Gundersen, 2008 ) and detraining ( Bruusgaard et al., 2010 ).

Myonuclei are not lost during atrophy. Time-lapse in vivo imaging of the same fibre segment before (day 0) and after 21 days of denervation. Note that the number of nuclei in the segment left of the neuromuscular endplate labelled in red is the same in spite of the pronounced atrophy. Scale bar, 50 μm. Adapted from Gundersen and Bruusgaard (2008) .
Although in vivo imaging represents direct observation of myonuclei, several papers (e.g. Alway et al., 2003a ; Ferreira et al., 2006 ; Pierce et al., 2008 ; Siu and Alway, 2005 ; Siu et al., 2005 ; Tang et al., 2000 ) have found markers for apoptosis in atrophying muscle homogenates and have interpreted this as apoptosis of myonuclei, but clearly this could reflect apoptosis of non-myonuclei.
There is also a large literature based on histological analysis using conventional staining techniques stating that nuclei are lost by apoptosis during atrophy (e.g. Adhihetty et al., 2007 ; Allen et al., 1997 ; Alway et al., 2003b ; Meneses et al., 2014 ; Tews et al., 1997 ; Yoshimura and Harii, 1999 ). However, with such a technique it is difficult to distinguish between nuclei of different cell types, in particular satellite cells, which are located under the basal lamina of the muscle fibres.
Newer studies have used antibodies against the myofibre cytoskeleton protein dystrophin to outline the fibre surface as a way to separate myonuclei from the nuclei of satellite and stroma cells. Even this literature has yielded conflicting results, and the precise rules for deeming a nucleus to be inside or outside the dystrophin ‘ring’ on cross-sections seems to be crucial for the result. Unfortunately, these rules are generally poorly described in the literature.
In our studies we have defined myonuclei as nuclei with their geometrical centre inside the inner rim of the dystrophin ring ( Bruusgaard et al., 2012 , , 2010 ; Bruusgaard and Gundersen, 2008 ; Gundersen and Bruusgaard, 2008 ). Using this definition, the averages are all below 1.7 mynuclei per fibre on 8–10 µm cross-sections. When the same operator in our laboratory evaluated the same sections, but also included nuclei with their geometrical centre on the dystrophin ring, the number of ‘myonuclei’ increased by 85% ( Bruusgaard et al., 2012 ), and it is questionable whether these were all myonuclei. Using the strict definition, however, we have essentially never observed apoptotic myonuclei under atrophy conditions ( Bruusgaard et al., 2012 , , 2010 ; Bruusgaard and Gundersen, 2008 ; Gundersen and Bruusgaard, 2008 ).
Others using dytrophin staining have also described myonuclear apoptosis and/or loss of myonuclei during atrophy ( Dupont-Versteegden et al., 2006 ; Guo et al., 2012 ; Leeuwenburgh et al., 2005 ; Oishi et al., 2008 ; Zhu et al., 2013 ). However, they reported a >30% higher number of defined myonuclei (>2.2 myonuclei per fibre) on 6–10 µm cross-sections ( Dupont-Versteegden et al., 2006 ; Leeuwenburgh et al., 2005 ; Oishi et al., 2008 ), suggesting that the population of nuclei included was considerably less selective than in our studies.
In addition to the problem of identifying myonuclei, the terminal deoxynucleotidyl transferase dUTP nick end (TUNEL) staining used to label apoptotic nuclei is prone to false positives ( Garrity et al., 2003 ; Pulkkanen et al., 2000 ). With a labelling incidence of 1.5 nuclei per section in normal control material from rat limb ( Gundersen and Bruusgaard, 2008 ), virtually no apoptotic myonuclei appeared upon denervation, while many apoptotic nuclei appeared in mononuclear cells ( Gundersen and Bruusgaard, 2008 ). In contrast, a report displaying seven-times higher incidences of TUNEL-positive nuclei in comparable control material reported a loss of myonuclei by apoptosis ( Dupont-Versteegden et al., 2006 ).
Several studies based on fibres isolated ex vivo after denervation have also concluded that myonuclei are not lost during atrophy ( Aravamudan et al., 2006 ; Bruusgaard et al., 2012 , , 2010 ; Duddy et al., 2011 ; Wada et al., 2002 ). Duddy et al. (2011) used cultured, live fibres and identified myonuclei very precisely using a muscle- specific reporter gene. Wada et al. (2002) and Bruusgaard et al. ( 2010 , 2012 ) isolated fibres by alkaline maceration. When Wada et al. (2002) compared this method with mechanical isolation, they found that mechanical isolation was unreliable because of both adhering non-myonuclei and loss of myonuclei from the fibres. This might explain why a loss of myonuclei has been reported after mechanical isolation ( Viguie et al., 1997 ). Similarly, Kawano et al. (2008) reported a loss of myonuclei after collagenase digestion, but their myonuclear counts were three to four times higher than those observed by in vivo imaging, suggesting that adherent cells were also a problem with this technique.
In conclusion, by direct observation with in vivo imaging one observes no loss of myonuclei during atrophy, and this is confirmed by observations of isolated single fibres ex vivo when contamination of non-myonuclei adhering to the fibres is minimized. These observations are also confirmed by the histological studies with the most conservative inclusion criteria for myonuclei. Similarly, the studies with the least risk of false positive TUNEL-labelling have failed to demonstrate apoptotic myonuclei. Thus, there is currently no compelling reason to believe that myonuclear apoptosis occurs under atrophy conditions. One might question whether a selective apoptosis of some nuclei within an intact syncytium ever takes place or is even possible. This does not exclude the possibility that whole fibres or fibre segments could be eliminated by apoptosis, but this is not relevant for the atrophy of pre-existing fibres.
What determines the number of myonuclei?
The conclusion that myonuclei are recruited during hypertrophy and not lost during subsequent atrophy seems to suggest that by going through repeated hypertrophy/atrophy cycles one could ‘pump up’ the number of nuclei indefinitely. This seemed implausible, and we ( Bruusgaard et al., 2012 ) therefore reinvestigated the fate of myonuclei in the hind limb suspension model where atrophy is induced by lifting up the hind part of rats by the tail and thereby unloading the hind legs. Using in vivo imaging, we observed that myonuclei were not lost during the resulting atrophy. When the muscles were reloaded by letting the animal back down again, the fibres displayed a 60% radial growth, and this growth was not accompanied by any increase in the number of myonuclei. Such growth also does not seem to require the presence of satellite cells ( Jackson et al., 2012 ). Thus, we suggest that hypertrophy can occur without new myonuclei, provided the myonuclear number is already high.
Based on data from human studies, the so-called ‘ceiling hypothesis’ has been put forward. It states that until a certain limit of hypertrophy is reached, hypertrophy can occur without recruiting new myonuclei ( Kadi et al., 2005 ). The limit has been defined as hypertrophy above a certain per cent (e.g. 17–36%) ( Kadi et al., 2005 ) or as an absolute myonuclear cytoplasmic domain volume that, when exceeded, triggers recruitment of myonuclei ( Petrella et al., 2006 , , 2008 ).
We suggest that the number of myonuclei not only reflects the current size of the fibre as implied by the ceiling hypothesis, but also the history of the fibre. Current data might fit a ‘peak pegging’ hypothesis, where the number of myonuclei found in a fibre represents the largest size the fibre has had in its history, and new myonuclei are only added if the fibre grows beyond that size. This would be analogous to a conventional minimum/maximum thermometer, where the mercury column pushes a peg upwards and leaves it at the highest temperature measured.
As concluded above, myonuclei seem to be recruited during overload hypertrophy, and not lost during subsequent atrophy (see particularly Egner et al., 2013 ). Thus, a previously hypertrophied fibre would differ from a naive fibre in having more nuclei as a ‘memory of its past’. Technically, the fibre displays hysteresis: the cyto-architecture depends on previous exposure. This observation led us ( Bruusgaard et al., 2010 ) to propose the model for the cell biology of muscle size regulation illustrated in Fig. 4 . In this model, previously untrained fibres are small with few nuclei; when subjected to a hypertrophic stimulus they acquire new nuclei through a ‘first training route’. These new nuclei precede the growth in time and might be causally related to the subsequent fibre enlargement. The end product is a large fibre with many nuclei. Upon subsequent detraining, the fibres maintain the elevated number of nuclei, but lose protein resulting in a small fibre with a high number of nuclei. If the fibre is re-trained from this state we suggest that a different re-training route can be followed, skipping the step of recruiting myonuclei. The next questions we asked were whether this re-training route is beneficial, and whether it is easier or faster than the first training route.

A new model for the cell biology of hypertrophy and atrophy. For naive fibres and preceding hypertrophic growth, myonuclei are recruited from satellite cells, temporarily reducing the myonuclear domain volume, leading to a large fibre with many myonuclei. Upon subsequent atrophy the myonuclei are maintained, leading to a small fibre with a high myonuclear density and small myonuclear domains. Such fibres can hypertrophy without recruiting new nuclei, and this re-training route seems to be faster than the first training route. The permanently higher number of myonuclei represents the muscle memory. Adapted from Bruusgaard et al. (2010) .
The re-training route is faster
To investigate whether there is a beneficial muscle memory, Egner et al. (2013 ) treated female mice with testosterone for 2 weeks. As illustrated in Fig. 5 , this led to an increase in both fibre size and the number of nuclei. Three weeks after drug withdrawal, the fibre size was back to normal, but the number of nuclei remained constant and high. Three months later (>10% of the mouse lifespan), the number of nuclei was still essentially maintained. When at this time the muscles were subjected to overload, the high-nuclei muscles grew by 36%, while the control group grew insignificantly by only 6%. After that, the groups grew in parallel, but the high-nuclei group remained larger for the duration of the 2-week overload experiment. Egner et al. (2013) concluded that the re-training route seems to allow faster growth than the first training route, and suggest that the memory storage mechanism is the number of nuclei.

Demonstration of beneficial muscle memory in mice. Schematic representation of an experiment ( Egner et al., 2013 ) demonstrating the encoding, storage and retrieval of muscle memory. The encoding occurred during a brief exposure of the animals to testosterone, which led to an increase in fibre size and the number of myonuclei. When the drug was removed, fibre size reverted to control levels of sham-treated animals, but the high number of myonuclei was retained (storage). When both the testosterone and the sham groups were subjected to overload 3 months later, the former group grew much faster than the latter group (retrieval).
How lasting is the muscle memory?
We have demonstrated that in mice, the memory effect lasts for >10% of the animal’s lifespan. In humans, the turnover of cells has been studied by utilizing the peak in 14 C availability after the post-war atmospheric testing of nuclear bombs ( Spalding et al., 2005 ). Intercostal muscle tissue was harvested from two individuals (age 37–38 years) and the 14 C content in genomic DNA indicated an average age of the nuclei of 15.1 years. For several reasons this is a low-end estimate of the possible lifespan of human myonuclei. First, approximately half of the nuclei in the tissue are in other cell types, mostly with a higher turnover. By comparison, in gut approximately 40% of the cells are epithelial with a turnover rate measured in days; when these were excluded the average lifetime of nuclei was increased by 50% in that tissue. Second, the measurement was from young individuals that had been growing for a significant part of their life and thus creating new myonuclei. Third, new myonuclei might have been created during relatively recent strength exercise.
It is possible that myonuclei are more or less permanent once created; for example, human brain cortical neurons appeared to be as old as the individual ( Spalding et al., 2005 ). For cardiomyocytes, 1% turn over annually at an age of 25 years, gradually decreasing to 0.45% at an age of 75 years. It was calculated that fewer than 50% of cardiomyocytes are exchanged during a normal lifespan ( Bergmann et al., 2009 ). In contrast to the brain and heart, however, muscle tissue is subjected to repair after damage with new nuclei from satellite cells. How much damage and repair normally accumulates over decades is, however, unknown. Thus, with current knowledge it is hard to be more precise than estimating the lifespan of myonuclei, and hence the muscle memory span, to somewhere between 15 years and a human lifetime.
Evolutionary considerations
One might speculate as to the evolutionary origin of muscle memory. Based on the original model ( Fig. 2 ), it was speculated that nuclei were lost during atrophy because they might be expensive to maintain. ‘Cost’ might be energy or space; muscle fibres are optimized with respect to packing contractile proteins. However, the cost of keeping a high number of nuclei in a small fibre has never been quantified, nor compared with the cost of eliminating and recreating myonuclei with ever-changing demands for muscle strength.
In the new model ( Fig. 4 ), the muscle memory might represent an adaptive mechanism to allow individuals from whom strength has been demanded in the past to more quickly rebuild muscle mass in the future, as an individual adaption or specialization. In this way, maintaining a constant high muscle mass is avoided, for example, during less labour-intensive seasons. As for the classical learning and memory of the brain, ‘experience’ becomes useful if the same task arises again.
Healthy ageing
Decline in muscle strength is a major health problem in the ageing western population ( Dutta and Hadley, 1995 ; Hughes and Schiaffino, 1999 ), and more than 50% of individuals currently fill the clinical criteria for frailty at ages >80 years ( Matthews et al., 2011 ). Hypertrophy induced by overload is greatly attenuated in older animals ( Alway et al., 2002 ; Carson et al., 1995 ), and the ability to generate new myonuclei is impaired ( Schultz and Lipton, 1982 ), possibly because of reduced notch signalling in the elderly ( Conboy et al., 2003 ; Conboy and Rando, 2005 ). The role of a similar nuclei-related muscle memory in humans should be investigated. Such knowledge might lead to public health advice for strength training to recruit new myonuclei in younger individuals, as these myonuclei might aid in maintaining muscle mass more easily in senescence.
Muscle memory was induced in mice by testosterone, and it was demonstrated that brief exposure to this hormone aided hypertrophy induced by overload long after the drug was removed ( Egner et al., 2013 ). If this is applicable to humans, it must have consequences for doping rules. According to Anti-Doping Norway, animal experiments on the permanency of recruited myonuclei ( Bruusgaard and Gundersen, 2008 ) have already contributed to increasing the exclusion time for doping offences from 2 to 4 years. Given the longevity of human myonuclei, this would not be sufficient to ensure that previous steroid use does not still give a competitive advantage when the exclusion time is over. The position of the World Anti-Doping Agency has been that no regulatory action should be taken before the muscle memory phenomenon is confirmed in humans. This raises interesting questions about the burden of proof for convicted cheaters. Nonetheless, if confirmed in humans, the possibility of a long-term muscle memory induced by a brief steroid exposure raises serious questions about the possibility of policing a drug-free sport.
Although there is evidence for the new model shown in Fig. 4 and for muscle memory induced by testosterone in rodents ( Fig. 5 ), it should also be demonstrated that muscle memory could be encoded by strength exercise without steroids. As for humans, although anecdotal evidence suggests the existence of training-induced muscle memory, this needs to be confirmed in controlled exercise studies.
Although the demonstrated long-lasting increase in the number of myonuclei is a logical epigenetic substrate for the memory phenomenon, it does by no means exclude other mechanisms. DNA and histone modifications are now widely accepted for gametogenesis and early cell differentiations. There is also emerging evidence that such mechanisms occur in somatic, fully differentiated cells such as muscle cells, and that they might play a role in the malleability of muscle phenotype in response to exercise ( Rasmussen et al., 2014 ).
K.G. is supported by the Research Council of Norway [grant 240374].
Email alerts
2023 jeb outstanding paper prize shortlist and winner.
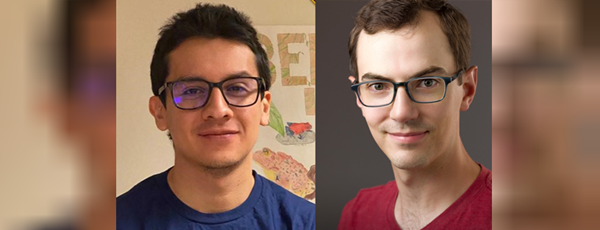
The JEB Editors are delighted to announce the shortlisted authors for the 2023 JEB Outstanding Paper Prize . Read the winning paper - Tiny spies: mosquito antennae are sensitive sensors for eavesdropping on frog calls - by Hoover Pantoja-Sanchez and Brian Leavell from Ximena Bernal's lab at Purdue University, USA.
JEB Science Communication Workshop for ECRs
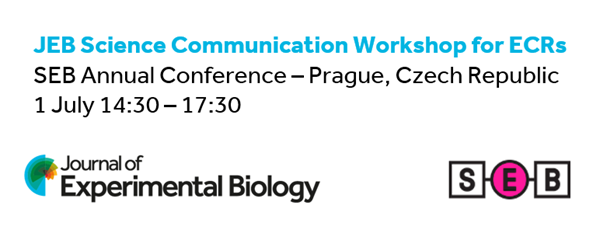
If you’re an early-career researcher interested in science communication and are attending the SEB Annual Conference in Prague this summer, come a day early and join the JEB Editors at a sci comm workshop to learn the key writing skills needed to promote your research to a broad audience beyond your peers (1 July at 14.30-17.30). Places are limited to 24 attendees, and applicants should apply through the SEB registration page by 30 April 2024.
Bridging the gap between controlled conditions and natural habitats in understanding behaviour
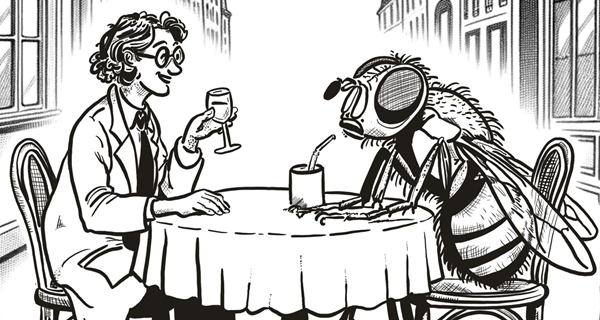
Novel technologies enable behavioural experiments with non-model species, in naturalistic habitats and with underexplored behaviours. In their Commentary , Scholz and colleagues discuss how to obtain a deeper understanding of the natural ecology and lifestyle of study animals.
Beluga metabolic measures could help save species
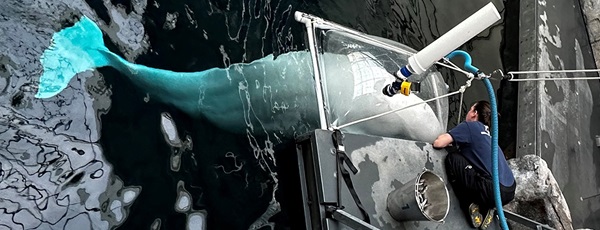
To help save animals from extinction, it’s important to understand what each species needs to survive. This led Jason John et al. to measure the metabolic rates of captive belugas to develop a ‘fish calculator’ showing that the whales need to eat ~23 salmon per day.
ECR Workshop on Positive Peer Review
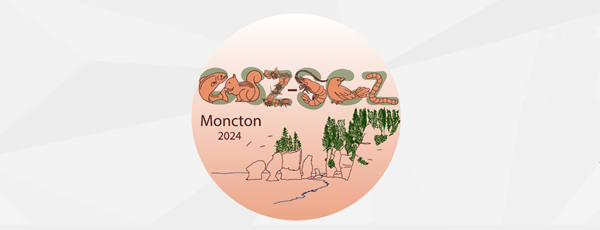
Are you an ECR looking for tips on how to write concise, astute and useful manuscript reviews? If so, join the JEB Editors at a 2-hour JEB-sponsored Workshop on Positive Peer Review at the Canadian Society of Zoologists annual meeting in Moncton on 9 May 2024 at 13.00-15.00. There are 25 spaces for ECRs and selection is first come, first serve. To sign up, check the ECR Workshop box when you register for the CSZ meeting.
Social media
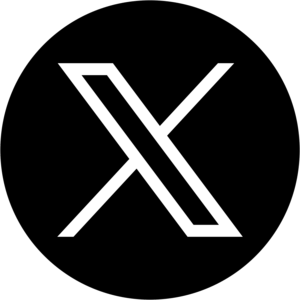
Other journals from The Company of Biologists
- Development
- Journal of Cell Science
- Disease Models & Mechanisms
- Biology Open
- Editors and Board
- Aims and scope
- Submit a manuscript
- Manuscript preparation
- Journal policies
- Rights and permissions
- Sign up for alerts
Affiliations
- Journal of Experimental Biology
- Journal Meetings
- Library hub
- Company news
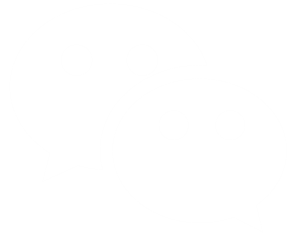
- Privacy policy
- Terms & conditions
- Copyright policy
- © 2024 The Company of Biologists. All rights reserved.
- Registered Charity 277992 | Registered in England and Wales | Company Limited by Guarantee No 514735 Registered office: Bidder Building, Station Road, Histon, Cambridge CB24 9LF, UK
This Feature Is Available To Subscribers Only
Sign In or Create an Account
Stanford researchers observe memory formation in real time

By Alan Toth
Why is it that someone who hasn’t ridden a bicycle in decades can likely jump on and ride away without a wobble, but could probably not recall more than a name or two from their 3rd grade class?
This may be because physical skills — dubbed motor memories by neuroscientists — are encoded differently in our brains than our memories for names or facts.
Now, a new study by scientists with the Wu Tsai Neurosciences Institute is revealing exactly how motor memories are formed and why they are so persistent. It may even help illuminate the root causes of movement disorders like Parkinson’s disease.
“We think motor memory is unique,” said Jun Ding , an associate professor of neurosurgery and of neurology. “Some studies on Alzheimer’s disease included participants who were previously musicians and couldn’t remember their own families, but they could still play beautiful music. Clearly, there’s a huge difference in the way that motor memories are formed.”
Memories are thought to be encoded in the brain in the pattern of activity in networks of hundreds or thousands of neurons, sometimes distributed across distant brain regions. The concept of such a memory trace — sometimes called a memory engram — has been around for more than a century, but identifying exactly what an engram is and how it is encoded has proven extremely challenging. Previous studies have shown that some forms of learning activate specific neurons, which reactivate when the learned memory is recalled. However, whether memory engram neurons exist for motor skill learning remains unknown.
Ding and postdoctoral scholars Richard Roth and Fuu-Jiun Hwang wanted to know how these engram-like groups of cells get involved in learning and remembering a new motor skill.
“When you’re first learning to shoot a basketball, you use a very diverse set of neurons each time you throw, but as you get better, you use a more refined set that’s the same every time,” said Roth. “These refined neuron pathways were thought to be the basis of a memory engram, but we wanted to know exactly how these pathways emerge.”
In their new study, published July 8, 2022 in Neuron , the researchers trained mice to use their paws to reach food pellets through a small slot. Using genetic wizardry developed by the lab of Liqun Luo , a Wu Tsai Neurosciences Institute colleague in the Department of Biology, the researchers were able to identify specific neurons in the brain’s motor cortex — an area responsible for controlling movements — that were activated during the learning process. The researchers tagged these potential engram cells with a fluorescent marker so they could see if they also played a role in recalling the memory later on.
When the researchers tested the animals’ memory of this new skill weeks later, they found that those mice that still remembered the skill showed increased activity in the same neurons that were first identified during the learning period, showing that these neurons were responsible for encoding the skill: the researchers had observed the formation of memory engrams.
But how do these particular groups of neurons take on responsibility for learning a new task in the first place? And how do they actually improve the animal’s performance?
To answer these questions, the researchers zoomed in closer. Using two-photon microscopy to observe these living circuits in action, they observed the so-called “engram neurons” reprogram themselves as the mice learned. Motor cortex engram cells took on new synaptic inputs — potentially reflecting information about the reaching movement — and themselves formed powerful new output connections in a distant brain region called the dorsolateral striatum — a key waystation through which the engram neurons can exert refined control over the animal’s movements. It was the first time anyone had observed the creation of new synaptic pathways on the same neuron population — both at the input and the output levels — in these two brain regions.
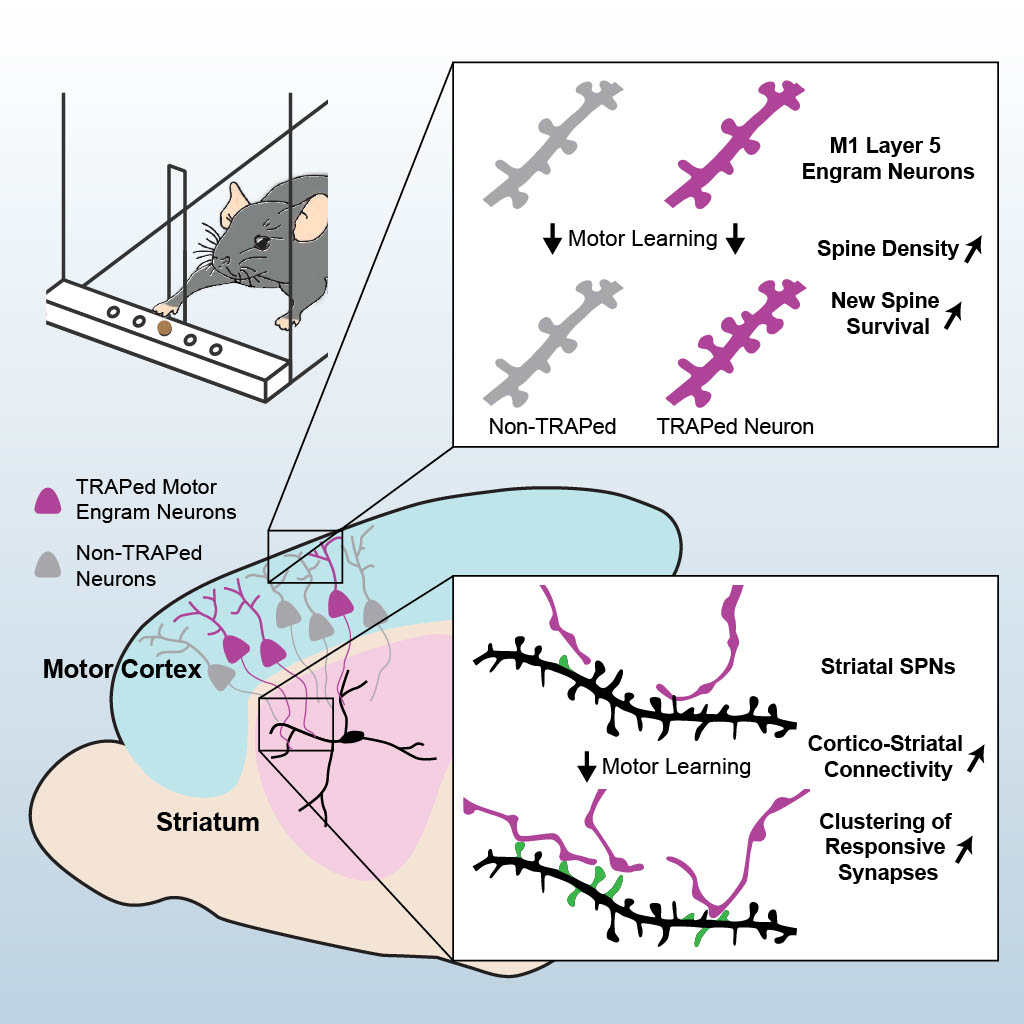
The ability to trace new memories forming in the mouse brain allowed the research team to weigh in on a long-standing debate about how skills are stored in the brain: are they controlled from one central memory trace, or engram, or is the memory redundantly stored across many different brain areas? Though this study cannot discount the idea of centralized memory, it does lend credibility to the opposing theory. Another fascinating question is whether the activation of these engram neurons is required for the performance of already learned motor tasks. The researchers speculated that by suppressing the activity of neurons that had been identified as part of the motor cortex memory engram, the mice probably still would be able to perform the task.
“Think of memory like a highway. If 101 and 280 are both closed, you could still get to Stanford from San Francisco, it would just take a lot longer,” said Ding.
These findings suggest that, in addition to being dispersed, motor memories are highly redundant. The researchers say that as we repeat learned skills, we are continually reinforcing the motor engrams by building new connections — refining the skill. It’s what is meant by the term muscle memory — a refined, highly redundant network of motor engrams used so frequently that the associated skill seems automatic.

Ding believes that this constant repetition is one reason for the persistence of motor memory, but it’s not the only reason. Memory persistence may also be affected by a skill being associated with a reward, perhaps through the neurotransmitter dopamine. Though the research team did not directly address it in this study, Ding’s previous work in Parkinson’s disease suggests the connection.
“Current thinking is that Parkinson’s disease is the result of these motor engrams being blocked, but what if they’re actually being lost and people are forgetting these skills?” said Ding. “Remember that even walking is a motor skill that we all learned once, and it can potentially be forgotten.”
It’s a question that the researchers hope to answer in a follow-up study, because it may be the key to developing effective treatments for motor disorders. If Parkinson’s disease is the result of blocked motor memories, then patients should be able to improve their movement abilities by practicing and reinforcing these motor skills. On the other hand, if Parkinson’s destroys motor engrams and inhibits the creation of new ones — by targeting motor engram neurons and their synaptic connection observed in the team’s new study — then a completely different approach must be taken to deliver effective treatments.
“Our next goal is to understand what’s happening in movement disorders like Parkinson’s,” Ding said. “Obviously, we’re still a long way from a cure, but understanding how motor skills form is critical if we want to understand why they’re disrupted by disease.”
The research was published July 8 in Neuron: https://doi.org/10.1016/j.neuron.2022.06.006
Study authors were Fuu-Jiun Hwang, Richard H. Roth, Yu-Wei Wu, Yue Sun, Destany K. Kwon, Yu Liu, and Jun B. Ding.
The research was supported by the National Institutes of Health (NIH) and National Institute for Neurological Disease and Stroke (NINDS); the Klingenstein Foundation's Aligning Science Across Parkinson’s initiative; and GG gift fund, the Stanford School of Medicine Dean’s Postdoctoral Fellowship; and Parkinson’s Foundation Postdoctoral Fellowship.
Related stories

Study proves 'muscle memory' exists at a DNA level
A study led by researchers at Keele University has shown for the first time that human muscles possess a 'memory' of earlier growth -- at the DNA level.
Periods of skeletal muscle growth are 'remembered' by the genes in the muscle, helping them to grow larger later in life.
The research, published in Scientific Reports , could have far-reaching implications for athletes caught using performance-enhancing muscle building drugs -- as the drugs could be creating long-lasting changes, making short-term bans inadequate.
Using the latest genome wide techniques, the researchers from Keele, along with the Universities of Liverpool John Moores, Northumbria and Manchester Metropolitan, studied over 850,000 sites on human DNA and discovered the genes 'marked' or 'unmarked' with special chemical 'tags' when muscle grows following exercise, then returns back to normal and then grows again following exercise in later life.
Known as epigenetic modifications, these 'markers' or 'tags' tell the gene whether it should be active or inactive, providing instructions to the gene to turn on or off without changing the DNA itself.
Dr Adam Sharples, the senior and corresponding author of the study and Senior Lecturer in Cell and Molecular Muscle Physiology at Keele University and his PhD student Mr Robert Seaborne explained:
"In this study, we've demonstrated the genes in muscle become more untagged with this epigenetic information when it grows following exercise in earlier life, importantly these genes remain untagged even when we lose muscle again, but this untagging helps 'switch' the gene on to a greater extent and is associated with greater muscle growth in response to exercise in later life -- demonstrating an epigenetic memory of earlier life muscle growth!"
The research has important implications in how athletes train, recover from injury, and also has potentially far-reaching consequences for athletes caught cheating.
Dr Sharples explained: "If an athlete's muscle grows, and then they get injured and lose some muscle, it may help their later recovery if we know the genes responsible for muscle 'memory'. Further research will be important to understand how different exercise programmes can help activate these muscle memory genes."
Mr Seaborne continued: "If an elite athlete takes performance-enhancing drugs to put on muscle bulk, their muscle may retain a memory of this prior muscle growth. If the athlete is caught and given a ban -- it may be the case that short bans are not adequate, as they may continue to be at an advantage over their competitors because they have taken drugs earlier in life, despite not taking drugs anymore. More research using drugs to build muscle, rather than exercise used in the present study, is required to confirm this."
- Fibromyalgia
- Chronic Fatigue Syndrome
- Muscular Dystrophy
- Human Biology
- Memory-prediction framework
- DNA microarray
- Limbic system
Story Source:
Materials provided by Keele University . Note: Content may be edited for style and length.
Journal Reference :
- Robert A. Seaborne, Juliette Strauss, Matthew Cocks, Sam Shepherd, Thomas D. O’Brien, Ken A. van Someren, Phillip G. Bell, Christopher Murgatroyd, James P. Morton, Claire E. Stewart, Adam P. Sharples. Human Skeletal Muscle Possesses an Epigenetic Memory of Hypertrophy . Scientific Reports , 2018; 8 (1) DOI: 10.1038/s41598-018-20287-3
Cite This Page :
Explore More
- Plastic Pollution Kills Ocean Embryos
- Most Massive Stellar Black Hole in Our Galaxy
- Coffee's Prehistoric Origin and It's Future
- Can Animals Count? New Rat Study
- A Single Atom Layer of Gold: Goldene
- Fool's Gold May Contain Valuable Lithium
- Exercise Cuts Stress-Related Brain Activity
- Microplastics Go from Gut to Other Organs
- Epilepsy Drug May Prevent Brain Tumors
- Evolution's Recipe Book
Trending Topics
Strange & offbeat.
Muscle memory and a new cellular model for muscle atrophy and hypertrophy
Affiliation.
- 1 Department of Biosciences, University of Oslo, Blindernveien 31, Oslo N0316, Norway [email protected].
- PMID: 26792335
- DOI: 10.1242/jeb.124495
Memory is a process in which information is encoded, stored, and retrieved. For vertebrates, the modern view has been that it occurs only in the brain. This review describes a cellular memory in skeletal muscle in which hypertrophy is 'remembered' such that a fibre that has previously been large, but subsequently lost its mass, can regain mass faster than naive fibres. A new cell biological model based on the literature, with the most reliable methods for identifying myonuclei, can explain this phenomenon. According to this model, previously untrained fibres recruit myonuclei from activated satellite cells before hypertrophic growth. Even if subsequently subjected to grave atrophy, the higher number of myonuclei is retained, and the myonuclei seem to be protected against the elevated apoptotic activity observed in atrophying muscle tissue. Fibres that have acquired a higher number of myonuclei grow faster when subjected to overload exercise, thus the nuclei represent a functionally important 'memory' of previous strength. This memory might be very long lasting in humans, as myonuclei are stable for at least 15 years and might even be permanent. However, myonuclei are harder to recruit in the elderly, and if the long-lasting muscle memory also exists in humans, one should consider early strength training as a public health advice. In addition, myonuclei are recruited during steroid use and encode a muscle memory, at least in rodents. Thus, extending the exclusion time for doping offenders should be considered.
Keywords: Atrophy; Hypertrophy; Muscle memory; Myonuclei; Satellite cells; Skeletal muscle; Strength training.
© 2016. Published by The Company of Biologists Ltd.
Publication types
- Research Support, Non-U.S. Gov't
- Hypertrophy
- Models, Biological
- Muscle, Skeletal / pathology*
- Muscle, Skeletal / physiopathology*
- Muscular Atrophy / physiopathology*
- Public Health
Thank you for visiting nature.com. You are using a browser version with limited support for CSS. To obtain the best experience, we recommend you use a more up to date browser (or turn off compatibility mode in Internet Explorer). In the meantime, to ensure continued support, we are displaying the site without styles and JavaScript.
- View all journals
- My Account Login
- Explore content
- About the journal
- Publish with us
- Sign up for alerts
- Review Article
- Open access
- Published: 23 November 2021
A comparative review of artificial muscles for microsystem applications
- Mayue Shi ORCID: orcid.org/0000-0001-8539-6873 1 &
- Eric M. Yeatman ORCID: orcid.org/0000-0003-0487-2693 1
Microsystems & Nanoengineering volume 7 , Article number: 95 ( 2021 ) Cite this article
15k Accesses
23 Citations
6 Altmetric
Metrics details
- Engineering
- Materials science
Artificial muscles are capable of generating actuation in microsystems with outstanding compliance. Recent years have witnessed a growing academic interest in artificial muscles and their application in many areas, such as soft robotics and biomedical devices. This paper aims to provide a comparative review of recent advances in artificial muscle based on various operating mechanisms. The advantages and limitations of each operating mechanism are analyzed and compared. According to the unique application requirements and electrical and mechanical properties of the muscle types, we suggest suitable artificial muscle mechanisms for specific microsystem applications. Finally, we discuss potential strategies for energy delivery, conversion, and storage to promote the energy autonomy of microrobotic systems at a system level.
Similar content being viewed by others
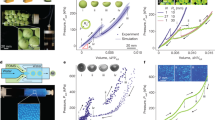
Shell buckling for programmable metafluids
Adel Djellouli, Bert Van Raemdonck, … Katia Bertoldi
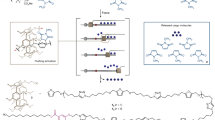
Force-controlled release of small molecules with a rotaxane actuator
Lei Chen, Robert Nixon & Guillaume De Bo
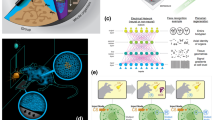
Collective intelligence: A unifying concept for integrating biology across scales and substrates
Patrick McMillen & Michael Levin
Introduction
With the miniaturization of electronics and the development of machine intelligence, various advanced electronic devices have been increasingly deployed in a wide range of living environments in the last few decades. Frequent and close human-machine interactions require devices that are soft, small-scale, lightweight, and power-efficient. Actuators, as a critical part of many electronic devices, also require these properties. However, conventional rigid actuators, such as electric motors and combustion engines, not only have safety problems but also suffer low overall efficiency and high cost 1 on the subcentimeter scale because of the mechanical impedance mismatch, significant friction loss, and complexity of fabrication.
Natural muscle is a soft biological actuator with outstanding driving capability, compliance, and additional functions such as self-healing. Specifically, skeletal muscles are composed of muscle fibers that are arranged in parallel between tendons, while muscle fibers are composed of myofibrils. A myofibril contains myosin filaments and actin filaments. The myofibril contracts when the actin filaments slide along the myosin filaments, energized by the hydrolysis of ATP (adenosine triphosphate). This process is under the control of neurons through the release of Ca 2+ . A relaxation process occurs due to the cessation of the interaction between actin and myosin when the concentration of Ca 2+ decreases. The movement is finally transferred to bones through tendons at the ends of the skeletal muscles. The detailed structure and molecular basis of skeletal muscles have been extensively explored, as shown in the existing literature 2 .
The excellent performance of natural muscles inspires researchers to realize such attractive functions with engineering materials and methods. Artificial muscles are a category of rapidly developing actuators that can mimic the properties and functions of natural muscle and are suitable for compliant interactions. G.M. Whitesides defined an actuator as a device that supplies mechanical energy to another device 3 . Based on this definition, the artificial muscle is clearly an actuator; however, it has several critical differences from conventional actuators, such as electric motors and pneumatic pistons, which have been widely used in industry. S.M. Mirvakili and I.W. Hunter defined that artificial muscles are a class of responsive materials and devices that can reversibly generate actuation, including contraction, expansion, or rotation within one component 4 . In our view, an artificial muscle has three typical features: small scale, low stiffness, and the capability of replicating a central function of natural muscle, i.e., generating actuation within an individual small device in response to an external stimulus. This stimulus can take many forms 4 , including an electrical signal 5 , pressure 3 , temperature 6 , a magnetic field 7 , 8 , etc.
Artificial muscles are particularly suitable for human-machine interaction applications because of their soft and tough mechanical properties, flexible drive mechanisms, low fabrication complexity, and acceptable efficiency at a miniature (subcentimeter) scale. In recent years, artificial muscles have developed rapidly, driven in part by new and improved materials, fabrication processes, and device structures. There has been an increasing amount of literature on artificial muscles based on a variety of actuation mechanisms, such as small-scale pneumatic/hydraulic artificial muscles 3 , 8 , dielectric elastomer (DE) artificial muscles 9 , ionic polymeric actuators 10 , micro piezoelectric actuators 11 , magnetic actuators 7 , and actuators based on shape memory alloys and polymers (SMAs and SMPs) 12 . A number of excellent reviews of various artificial muscles have been published in recent years. For example, Mirvakili et al. 4 reviewed various actuation mechanisms for artificial muscles and their mechanical properties. Hines et al. 13 summarized and discussed a variety of soft actuators categorized by different driving stimuli.
This study aims to provide a comparative review with practical opinions on the application and development of artificial muscles in microsystems based on previous reviews and some critical developments more recently. Specifically, the first aim of this paper is to review recent research into artificial muscles. We provide an overview of artificial muscles based on six important actuation principles, as shown in Fig. 1 , and especially focus on the progress over the past five years. Second, in this review, we both summarize the properties of various actuation mechanisms and emphasize a systematic comparative analysis of electrical and mechanical properties. Parameters significant for potential applications are highlighted, such as modulus, maximum stroke, response time, and power efficiency. The pros and cons of different kinds of artificial muscle are analyzed and compared. According to these analyses, we suggest suitable artificial muscle types for specific applications based on their unique electrical and mechanical properties. Finally, to realize highly efficient energy management, we discuss some methods involving energy delivery, storage, and conversion. This is especially useful for pursuing energy autonomy in microrobotic systems.
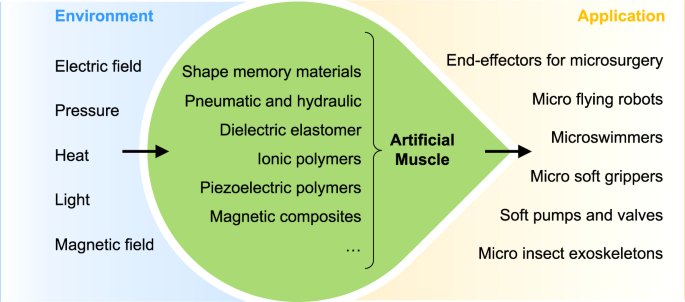
Artificial muscles are responsive to various environmental stimuli. They can generate actuation with high compliance for different microsystem applications
Artificial muscles with various actuating mechanisms
Many stimuli and mechanisms that drive artificial muscles have been investigated in recent years. According to different stimuli, artificial muscles can be categorized as thermoresponsive 6 , 14 , electrically responsive 15 , 16 , 17 , magnetically responsive 7 , 18 , 19 , photoresponsive 20 , chemically responsive 21 , and pressure driven 22 , 23 . Furthermore, some artificial muscles are multistimuli responsive 24 . The multiresponsive mechanism effectively increases the flexibility and adaptability of an artificial muscle but requires complex control of the device and ambient environment. According to specific actuating mechanisms, artificial muscles can be classified as SMAs and SMPs, DEs, ionic polymer-metal composites (IPMCs), pneumatic, hydraulic actuators, etc. This classification method is preferred in our review because it emphasizes the physical essence applied to generate actuation and thus can provide clear guidance for technical improvement. In this section, we aim to summarize properties and recent progress in six common actuating mechanisms.
SMAs and SMPs
The shape memory effect describes a phenomenon in which a material can be deformed and fixed into a temporary shape, after which recovery of the original shape can be realized by an external stimulus 25 . There are one-way and reversible (two-way) shape memory effects; some shape memory materials even have multishape memory properties 26 . The one-way shape memory effect allows one-way recovery from the temporary shape, while the reversible effect allows the material to be deformed between two shapes. The shape memory effect has been found in some alloys 27 , 28 , ceramics 29 , 30 , and polymers 25 , 31 , 32 . Among them, SMAs have been studied extensively since the first discovery in 1932 33 . W. Buehler and F. Wang discovered and developed the famous NiTi-based SMA (nitinol) in 1962. At present, NiTi-based SMAs are still the most preferable in applications compared to other SMAs, such as iron-based and copper-based SMAs 34 . Although iron-based and copper-based SMAs are low-cost and commercially available, their poor stability and thermomechanical performance often limit their further application 4 , 27 .
The shape memory effect in alloys originates from a reversible phase transition when the temperature varies. In the process of a phase transition, the SMA has three different crystal structures: twinned martensite, detwinned martensite, and austenite 27 , 35 . A typical one-way shape memory process can start from the twinned martensite at low temperatures. With mechanical loading, the twinned martensite transforms to detwinned martensite with deformation (deformed martensitic phase). Then, when the deformed SMA is heated to a temperature higher than the austenite start temperature ( A s ), it gradually recovers to its original shape and transforms to austenite. In this step, conductive SMAs can be easily driven with Joule heat. Finally, after a cooling process, the austenite returns to the twinned martensitic phase with the original shape.
The most significant limitation for SMAs is their low working frequency, which is caused by the low heat transfer rate, especially in the cooling stage. Several strategies have been explored to enhance heat transfer. For example, reducing the diameter of SMA fibers has proven beneficial due to an increase in the specific surface area 36 . Interestingly, this suggests that a microactuator based on an SMA could perform at a higher operating frequency than mesoscale devices. Moreover, heat convection has been studied to increase the response speed. An et al. provided a summary of relevant methods in ref. 27 .
In recent years, the soft nature of SMPs has attracted increasing attention. SMPs are particularly promising as artificial muscles because they show similar properties in several aspects to real muscle, namely, low mass density, low elastic modulus, and high shape recovery rate. Moreover, SMPs such as polylactic acid and polycaprolactone are biocompatible and biodegradable, which makes them particularly suitable for biomedical applications. The recovery time is often on the level of 1–100 s, and a typical produced force ranges from 1 to 100 MPa.
A one-way SMP can be first deformed at an elevated deformation temperature ( T d ), after which the deformed shape can be fixed with shape fixity ( R f ) while cooling. This temporary deformation will be released with shape recovery ( R r ) by heating the material to a recovery temperature ( T r ) 26 . For a reversible SMP, the programming process is similar. In ref. 25 , Lendlein and Gould provided a detailed discussion of the macroscale and molecular-scale mechanisms of an SMP. Briefly, the chain segments in the polymer are crosslinked through netpoints chemically and physically. These netpoints control the permanent shape at the macroscale. The temporary shape is determined by the additional reversible netpoints, which are formed during the programming process. These factors limit the recovery of oriented chain segments after programming, therefore fixing the temporary shape. If there is more than one phase transition temperature for different domains, then the SMP will have multiple shape memory effects 37 , 38 . A reversible shape memory effect is critical for soft actuators, but most conventional SMPs are one-way shape memory materials. The first reversible bidirectional SMP actuator was developed by Behl et al. 39 , 40 . In contrast to a one-way SMP, which has a “frozen” state at low temperature, deformation in the direction of orientation occurs in a reversible SMP during the cooling process due to a crystallization-induced elongation of the oriented segments associated with the lower melting temperature 40 .
Shape memory artificial muscles have been used for various applications 41 , 42 . Kim et al. used a NiTi spring as the muscle of an earthworm-like soft robot 43 . The spring structure was fabricated by winding the NiTi fiber (100 μm) around a core wire (200 μm). The spring-shaped muscle fibers are arranged in two directions to mimic the longitudinal muscle and circumferential muscle of the earthworm. In a further experiment, the robot exhibited a 50% contraction and an energy density of 1226 J/kg. Lee et al. developed microscale NiTi actuators with a diamond-shaped frame structure fabricated by focused ion beam (FIB) milling 44 (Fig. 2a ). When driven by laser heating, these actuators demonstrated a maximum strain of 60%, which was much higher than that of conventional bulk NiTi alloy (strain < 8% 27 ). Notably, these actuators are able to operate at high frequencies. Because of its extremely small volume, their device had a low thermal capacitance and a high specific surface area, which led to a very fast thermomechanical response up to 1.6 kHz.
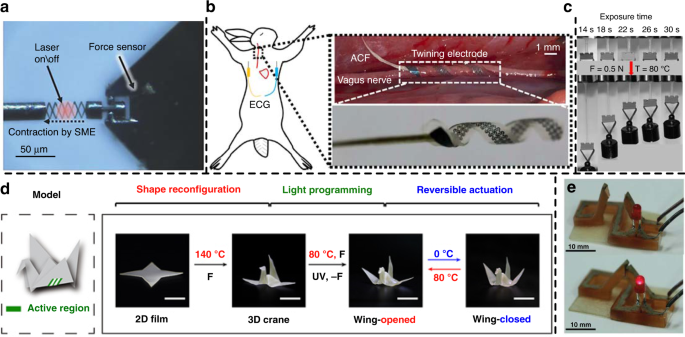
a SMA microactuator with diamond-shaped frame structures (1 µm in thickness) in the laser irradiation test 44 . Copyright 2018 by Wiley. Reprinted with permission. b Schematic diagram of twining electrodes using SMP for vagus nerve stimulation and recording of ECG (left), and photos of an implanted twining electrode (inner diameter of 1 mm) on the vagus nerve (right) 49 . Reproduced from ref. 49 (2019, CC BY 4.0). c 4D printed SMP with variable modulus 52 . Copyright 2019 by ACS. Reprinted with permission. d Programming of an artificial crane using thermo and photoreversible SMP 50 . Reproduced from ref. 50 (2018, CC BY 4.0). e 3D printed SMP switch with silver conductive ink 51 . Copyright 2016 by Wiley. Reprinted with permission
Because of the transparency of some SMPs, they have been studied as optical components in recent years. For example, an SMP light valve array was developed by Xu et al. 45 . The microstructured SMP was formed by single-step compression molding against a polydimethylsiloxane (PDMS) mold and then compressed against a flat mold at ~100 °C to form a temporary flat shape with a transmittance of ~50%. When heated by an indium tin oxide array with Joule heat on a transparent substrate, the microstructure could be selectively recovered, and the transmittance decreased to less than 0.8% after recovery. Another study reported recently by Zhang et al. shows that SMPs with submicrometer structures had more possibilities in displaying images with colors 46 .
SMPs can also be applied to realize the controllable deformation of structures. Complex geometries and locking assemblies with sequential folds have been realized using SMPs 47 , 48 . Figure 2b presents a biomedical application of the controllable deformation 49 . The SMP was used to form a twining electrode to realize vagus nerve stimulation and a recording of ECG. Figure 2d presents the programming and operating process of an artificial crane 50 with an SMP, which is programmable with thermo and photoreversible bonds. The wings showed reversible actuation while the temperature varied between 0 and 80 °C. 3D printing has been a flexible method for the fabrication of SMP actuators. Figure 2e presents a 3D printed SMP switch with silver conductive ink 51 . This actuator can switch between on and off states at different temperatures. Figure 2c presents 4D printed SMPs (there is an additional dimension of time) with different moduli and a hanging 500 g weight at 80 °C 52 . Through control of the exposure time of digital light in a single printing run, the SMP actuator has variable mechanical properties in different parts, which allows complex actuation behavior.
In addition, there has been considerable progress in materials research. In 2010, Xie found a tunable multishape memory effect in a perfluorosulfonic acid ionomer (PFSA) belt 26 . After this, Luo et al. developed triple-shaped polymeric composites 38 . Ze et al. reported that a magnetic SMP contains magnetic particles of Fe 3 O 4 and NdFeB 53 . The Fe 3 O 4 particles were used for shape locking and unlocking through inductive heating under an alternating current (AC) magnetic field. NdFeB particles provide programmable deformation under a driven magnetic field. Gurevitch et al. showed that by using SMP foams, the artificial muscle can operate with a very low elastic modulus 54 .
Carbon-based shape memory composites have been explored to further improve the performance of artificial muscles. By synthesizing carbon-based conductive shape memory composites 55 , 56 , thermoelectrical control with Joule heating can be realized conveniently. Moreover, SMP/carbon nanotube (CNT) composites can be driven by the thermal effect of microwaves, although a pure SMP had no response to microwaves 57 . In addition, the mechanical properties can be adjusted using carbon nanomaterials in the composites. Yuan et al. compared polyvinyl alcohol (PVA), PVA-CNT, and PVA-graphene oxide nanocomposite fibers as rotational microactuators 58 . Although the maximum tensile strain was below 30%, high torque with large angles of rotation was achieved when inserting twists into nanocomposite fibers.
Pneumatic and hydraulic artificial muscles
Pressure-based artificial muscles have been developing rapidly toward soft robotic applications. Equipped with pneumatic and hydraulic artificial muscles, soft robots can provide excellent compliance and safety for interactive applications. Typically, pressure-based artificial muscles can produce forces ranging from 0.01 to 100 MPa, while a typical stroke is 10–100%. The effective modulus of hydraulic artificial muscles is generally higher than that of pneumatic artificial muscles, which means that hydraulic muscles tend to generate higher force. Although conventionally, pressure-based actuators are less preferred in microsystems than other mechanisms such as electroresponsive and thermoresponsive artificial muscles 13 , recent studies have shown that pressure-based micro artificial muscles can also provide excellent force and stroke 59 , 60 . Some advanced fabrication processes have been studied and have contributed to the miniaturization of pneumatic and hydraulic artificial muscles. For instance, the use of 3D printing can provide extra freedom for their design and fabrication 22 , 61 , 62 . Laser cutting can be used for the 2D fabrication of thin pneumatic artificial muscles with high precision 63 . B. Gorissen explored a high-quality reactive ion etching process for PDMS flexible fluidic microactuators 64 . Several systematic reviews of micro pressure-based artificial muscles have been undertaken 13 , 65 .
Pressure-based artificial muscle has been widely used in emerging soft robots. The boundary between soft robots and artificial muscle research is fuzzy in some cases. These studies usually exhibit some overlap; for example, an artificial muscle can work as an end effector and have a critical influence on the performance of a soft robot. However, the research into soft robotics generally tends to emphasize a systematic architecture; thus, it involves more control strategies and practical applications 66 . Alternatively, artificial muscle research mainly focuses on the innovation and improvement of material and device structure designs 4 .
The structural design has a significant influence on the performance of artificial muscles, such as the strain and produced force. In general, pressure-based artificial muscles consist of elastic chambers and structural constraints. The variation in pressure results in directional deformation. The structural constraints limit the deformation of the actuation to a single dimension to generate extension/contraction, bending, or torsion under control. McKibben muscles with elastic tubes and woven fabric sleeves are classic and have been well studied. The woven and knitted fabrics can also be embedded in the elastomer. These fabrics can stand high pressure up to several hundred kilopascals and produce strains greater than 100%, although the delay and friction loss between the fabric and elastomer is relatively high. Other than fabric-based muscles, pneumatic soft actuators using low-modulus rubbers and multiple air chamber structures can provide compliant and dexterous actuation by controlling the chambers separately, but the produced force is relatively low. Some compliant structures, such as corrugated, folded, and wrinkled structures, can be used with harder materials so that these actuators can achieve both high force output and ultralarge deformation. These actuators have attracted much attention in recent years.
The development of soft, small and portable power sources is a major challenge for pneumatic artificial muscles and soft robots 66 . Wehner et al. compared and analyzed several pneumatic energy sources for soft robotics 67 . These authors found that microcompressors had a relatively high capacity but showed low maximum pressure and flow rates. Combustion provided a high energy density, but the peak forces were too high for many soft robots. Cylinders of high-pressure fluid have limited capacity; therefore, the operating time is limited. Monopropellant decomposition (hydrogen peroxide) could provide power with a simple structure but still required system-level development. Nevertheless, some efforts have been made to develop autonomous small soft robots with onboard energy sources 22 . In addition to the power sources mentioned above, electrolysis has also been used to drive diaphragm actuators, as reported by Pang et al. 68 . Figure 3b presents entirely soft, autonomous robots powered by catalytic decomposition of an onboard monopropellant fuel supply 69 .
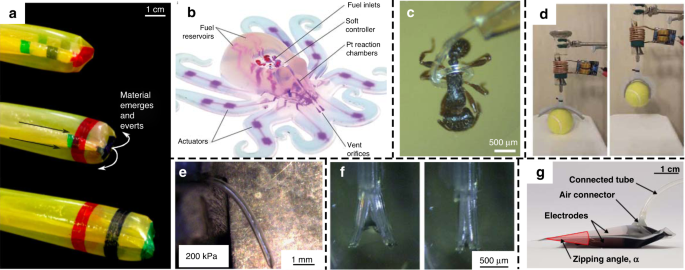
a A soft pneumatic actuator that can extend from the tip 71 . Copyright 2017 by AAAS. Reprinted with permission. b A fully soft autonomous robot 69 . Copyright 2016 by Springer Nature. Reprinted with permission. c A PDMS micro tentacle with spiral bending capability 60 . Reproduced from ref. 60 (2019, CC BY 4.0). d An artificial gripper using magnetically induced liquid-to-gas phase transitions 73 . Copyright 2020 by AAAS. Reprinted with permission. e A flexible fluidic microactuator 64 . Reproduced from ref. 64 (2016, CC BY 4.0). f Soft microfingers for cellular aggregate manipulation 75 . Reproduced from ref. 75 (2016, CC BY 4.0). g Soft electropneumatic pumps 76 . Copyright 2021 by AAAS. Reprinted with permission
Pneumatic artificial muscles can generate linear, torsional, and bending actuation, but twisting devices have been less studied than others. Gorissen et al. reported a flexible pneumatic twisting artificial muscle, which provided a bidirectional rotation of 6.5°/mm at a pressure of 178 kPa 70 . In addition, some effective configurations to provide elongation have been explored recently. For example, Hawkes et al. developed a soft pneumatic actuator that can extend from the tip of a body (Fig. 3a ) 71 . It has an onboard sensing capability to realize active direction control and is especially useful for navigation in narrow spaces. Blumenschein et al. studied a similar soft tip-extending structure for deploying antennas 72 . Miniaturization is challenging for pressure-based artificial muscles, but many studies have been carried out. Figure 3c shows a micro tentacle that can provide spiral bending actuation and hold an ant 60 . For pressure-based actuators, the produced force is proportional to the actuator cross-sectional area 13 . This means that for microscale artificial muscles, the produced force could be relatively low, but it could be a special opportunity for micromanipulation of some microscale biomaterials (such as devices shown in Fig. 3c , f). Pneumatic artificial muscles have been applied as soft grippers in many studies. For example, Mirvakili et al. presented an artificial pneumatic gripper (Fig. 3d ) using magnetically induced liquid-to-gas phase transitions so that bulky peripheral pumps were not needed. The volumetric expansion in the phase transition led to a significant pressure change that can be used for pneumatic artificial muscle 73 . It provided strains of up to 20% within 10 s. Song et al. reported a soft gripper with microfibrillar adhesives on a soft deformable membrane 74 . The soft backing makes the gripper suitable for manipulating parts with complex shapes by actively stretching the soft membrane. Gorissen et al. developed a high-performance etching process for PDMS based on SU8 masks, which were used for flexible fluidic microactuators, as shown in Fig. 3e 64 . Konishi and coauthors developed PDMS-based soft microfingers (Fig. 3f ) for pinching and releasing cellular aggregates (200 μm in diameter) 75 without obvious damage when applying up to 1 mN of restoring force. Diteesawat et al. developed soft electropneumatic pumps employing an electrostatic force and a dielectric fluid, which are only 1.1 mm in thickness and 5.3 g in weight (Fig. 3g ) 76 .
Dielectric elastomer
Electroactive polymers (EAPs) form a class of polymers that can respond to an electric field by generating mechanical motion 77 . These polymers can be simply divided into two categories: field-activated EAPs (such as DEs and piezoelectric polymers) and ionic EAPs (such as IPMC and ionic gels) 9 . In this section, we will first discuss the progress of a DE artificial muscle, which is also known as a DE actuator or DEA.
During the past two decades, DE artificial muscles have attracted increasing attention since R. Pelrine et al. reported the large actuation strain and fast response of acrylic and silicone elastomers in the late 1990s 9 , 15 , 78 because of their fast response, large strains, fracture toughness, and power-to-weight ratios compared to a natural muscle. The basic structure of DE-based artificial muscles can be considered to be a deformable capacitor. This field-actuated pressure across the DE can be simply written as p = ε 0 ε r E 2 , 14 , where ε 0 is vacuum permittivity, ε r is the relative dielectric constant of the DE, and E stands for the applied electric field in the DE. Under low strain conditions, the thickness strain s z can be approximately expressed as s z = − ε 0 ε r E 2 / Y , where Y is the apparent elastic modulus of the DE.
According to previous research 79 , the viscoelasticity and dielectric strength of the DE artificial muscle need more attention to further improve the actuation performance and prevent it from failing. The viscoelasticity of polymer materials causes significant viscous impedance at high frequencies, which limits the speed of the electromechanical response of DEs. A previous study has shown that the viscoelasticity is induced by the limited rotational freedom of the polymer chains and this viscoelastic loss could be reduced by adding plasticizer into the DE 80 . In addition, the typical driving voltage for the DEA varies from a few hundred to several thousand volts. High voltage leads to high strain, but the maximum voltage is often limited by the electromechanical instability of the elastomer. To decrease the required driving voltage for a required strain level, the dielectric constant could be increased by introducing functional fillers to DEs 81 , 82 . An example realized both a high dielectric constant and a high breakdown field using conductive polyaniline (PANI) particles encapsulated into an insulating polymer shell before dispersion, as reported by Molberg et al. 83 .
Many device-level and system-level improvements have been achieved over the last few years. Duduta et al. demonstrated a high-performance soft composite DE artificial muscle design consisting of strain-stiffening elastomers and CNT electrodes. The peak energy density reached 19.8 J/kg 5 . By applying self-healing elastomers, DE devices are able to realize the capability of self-healing 84 , 85 , 86 , which is a feature of natural muscle. Figure 4a shows a self-healing DE artificial muscle reported by Li et al. 84 . The healing process could occur at temperatures as low as −20 °C. Madsen et al. presented a self-healing DE with ionically crosslinked silicone 85 . Two system-level demonstrations are given in Fig. 4b , c. In Fig. 4b , Ji et al. used low voltage stacked DEAs in soft untethered and autonomous legged robots. The insect-scale device can move at 30 mm/s 87 . Figure 4c presents the structure and controlled flight of a microrobot driven by a central DE artificial muscle and related transmission components 16 .
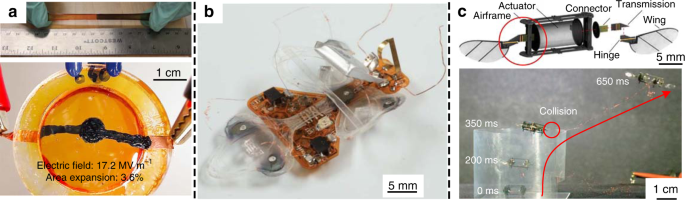
a A highly stretchable self-healing elastomer 84 . Copyright 2016 by Springer Nature. Reprinted with permission. b An insect-scale robot driven by low voltage stacked DE artificial muscles 87 . Copyright 2019 by AAAS. Reprinted with permission. c Controlled flight of a microrobot using DE artificial muscles 16 . Copyright 2019 by Springer Nature. Reprinted with permission
Ionic polymeric artificial muscle
Another important class of EAPs is ionic EAPs, which work based on the migration of cations and anions in an electric field. IPMCs are typical ionic polymeric artificial muscles consisting of an ion-conducting polyelectrolyte membrane sandwiched between flexible electrodes 13 , 88 . The polyelectrolyte membrane contains strong ionic groups such as sulfuric acid attached to the backbone of a stable polymer 89 . These highly ionic clusters provide mobile ions. In most cases, anions are fixed on the polymer backbone, while mobile hydrated cations transport to the cathode under an applied electric field, which results in unbalanced stress in the membrane and bending toward the anode direction.
Unlike DE artificial muscles, IPMCs can provide high actuation bending strain in response to a much lower voltage, usually from 1 to 5 V 4 , 89 , 90 , although electrolytes with mobile ions are required 90 . The low operating voltage is a significant advantage that allows many biomedical and MEMS applications. However, early IPMCs had to operate in an aqueous environment, so the electrolysis of water molecules and evaporation in open-air significantly limited their performance. Ionic liquids (ILs) have low volatility, wide potential windows, and high ionic conductivity, which can effectively improve the response speed and stability of IPMCs in open air 90 . Another challenge for IPMCs is that the fabrication process of metal electrodes needs to be optimized carefully to balance the flexibility, conductivity, and robustness. The advent of conductive carbon nanomaterials provides a promising alternative option for flexible electrodes 91 , 92 , 93 .
Kim et al. reported a high-performance durable IPMC based on single-ion conducting polymers with single-wall CNT electrodes on both sides (Fig. 5a ) 94 . This IPMC could provide millimeter-scale displacement under a voltage of only 1 V, while the response time was on the level of tens of milliseconds. This study shows an effective path to improve IPMC artificial muscles by introducing new ionic polymers. In addition, the response times of IPMCs are limited largely by the low mobility of ions at the electrode interface and across the electrode body. To solve this problem, Umrao et al. explored MXene composites as effective flexible electrodes (Fig. 5b ) 95 . Their device shows bending strains of 1.37% under sinusoidal input voltages of 1 V and 0.1 Hz. In addition, both of the above two studies realized actuation without back relaxation. Back relaxation is a main disadvantage of IPMCs under DC voltage, which means that the IPMCs will slowly relax after reaching a peak displacement. Figure 5d shows a graphdiyne-based electrochemical actuator 96 with a high electromechanical transduction efficiency of up to 6.03%. The verified alkene-alkyne complex transition effect is involved in this graphdiyne-based ionic polymeric actuator.
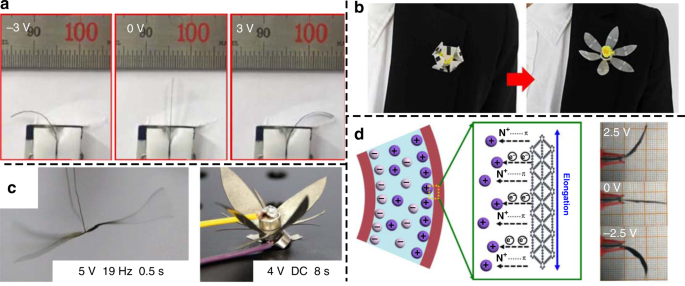
a Low driving voltage IPMC using single-ion conducting polymers 94 . Reproduced from ref. 94 (2016, CC BY 4.0). b IPMC actuator using MXene electrodes 95 . Copyright 2019 by AAAS. Reprinted with permission. c IPMCs are demonstrated to mimic a flapping-wing dragonfly and a flower using AC and DC power sources 97 . Copyright 2019 by Wiley. Reprinted with permission. d Graphdiyne-based IPMC actuator with an electromechanical transduction efficiency of up to 6.03% 96 . Reproduced from ref. 96 (2018, CC BY 4.0)
High-performance IPMC artificial muscles have been applied in biomimetic soft robots. As shown in Fig. 5c , Ma et al. reported a Nafion IPMC with homodispersed metal electrodes 97 , which was able to mimic the fast responsive flapping wings of a dragonfly under an AC voltage of 19 Hz, and an artificial flower under a DC voltage. In addition, Must et al. presented an IPMC composed of activated carbon-based electrodes and an IL 98 . With an onboard lithium battery, the untethered biomimetic inchworm could crawl on a smooth surface. Other studies involve applications such as robotic fish 99 .
In addition to IPMC artificial muscles, the improvement of hydrogels in recent years 100 , 101 makes ionic artificial muscles based on hydrogels particularly promising because of their outstanding compliance and biocompatibility. Yang and Suo reviewed ionotronic devices, including artificial muscles and electronic skins, based on ionic hydrogels 102 . Carbon nanotubes and graphene can also be used to form ionic artificial muscles 90 . These devices operate based on the electrostatic repulsive force between like charges injected into the actuating nanotube 103 . This kind of actuator often has low strains (<2%) because of the high stiffness of CNTs.
Piezoelectric artificial muscles
Piezoelectric materials have been widely used in microactuators for a long time. These materials have many applications, such as microlens actuators 104 , rotary 105 and stepping linear actuators 106 , micropumps 107 , and microgrippers 108 . Previous research has been reviewed extensively in refs. 109 , 110 .
Piezoelectric artificial muscles have many advantages, including a simple structure benefiting miniaturization, a relatively high power density at a small scale, and outstanding efficiency. In addition, the improvement of the fabrication process provides extra freedom for the design of micro piezoelectric actuators, such as the 3D printing of piezoelectric materials with designed anisotropy reported by Cui et al. 111 . However, the main challenges for piezoelectric artificial muscles include high stiffness, small strain, and relatively high operating voltage, which are incompatible with artificial muscle applications requiring compliant interaction. The stiffness, or the mechanical impedance, can be decreased by applying soft piezoelectric materials (such as piezoelectric polymers, composites, and other organic piezoelectric materials 112 ) and introducing compliant structures 113 . Although soft piezoelectric materials have been widely studied for energy harvesting 114 , 115 and sensing 116 , 117 , 118 , at present, there have been fewer studies on actuators based on these materials. Some examples include insect-scale soft robots using curved piezoelectric poly(vinylidene fluoride) (PVDF) films 119 , 120 . Another cantilever actuator using a PVDF bimorph 121 was reported by Liu et al. Compliant structures can help to adjust the output strain and force 122 . Vanderborght et al. provided a classification of reported variable stiffness/impedance actuators 123 . Although the actuation strain of piezoelectric actuators is low in general, it can be enlarged by introducing proper amplification mechanisms. For instance, an amplified piezoelectric actuator based on a piezo-hydraulic pump has been developed recently 124 . Kiziroglou et al. recently reviewed strategies and corresponding performances for micromotion amplification 125 . Figure 6c shows a high-precision millimeter-scale Delta robot 126 . The robot is driven by three independent piezoelectric bending actuators and a transmission linkage system. It can be operated in a 7.01 mm 3 workspace with a high precision of ~5 µm. In Fig. 6d , Suzuki and Woods developed a dexterous origami miniature manipulator with a remote center of motion for minimally invasive surgery, consisting of piezoelectric linear actuators and a pop-up parallelogram structure 127 . The motion range of the end effector could be effectively controlled by changing the leverage structure.
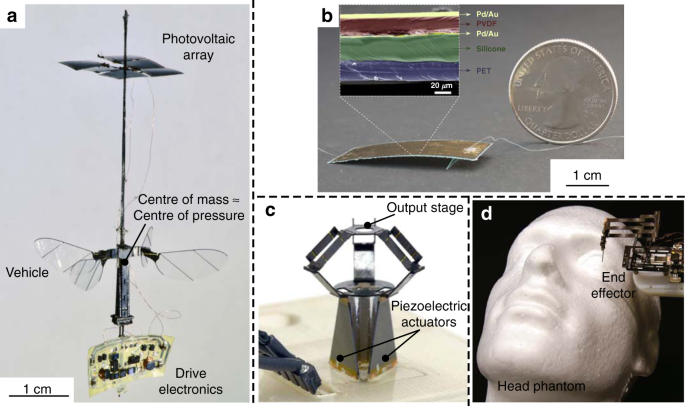
a An untethered piezoelectric aerial vehicle powered by a photovoltaic array 128 . Copyright 2019 by Springer Nature. Reprinted with permission. b Fast-moving soft robot based on PVDF 119 . The motion is in an oscillatory center of mass trajectory pattern similar to a cockroach. Copyright 2019 by AAAS. Reprinted with permission. c A millimeter-scale Delta robot driven by three piezoelectric bending actuators 126 . Copyright 2018 by AAAS. Reprinted with permission. d Origami-inspired miniature manipulator with a remote center of motion for microsurgery 127 . Copyright 2020 by Springer Nature. Reprinted with permission
Some system-level progress has been achieved in recent years, as shown in Fig. 6 , which suggests the potential toward high-performance autonomous microrobots based on piezoelectric artificial muscles. For example, piezoelectric actuators have been used in flapping-wing microscale aerial vehicles 17 , 128 . Figure 6a shows an untethered flapping-wing microscale aerial vehicle based on alumina-reinforced PZT actuators powered by a photovoltaic array 128 . The whole system was only 259 mg, and the power consumption was 110–120 mW. This vehicle shows the ability of untethered flight with an additional payload capacity. In addition, an autonomous-legged microrobot with RF communication and an onboard battery was reported by Goldberg et al. 129 . This device realized quick straight-line locomotion at 17.2 cm/s (3.8 body lengths per second). Figure 6b is a biomimetic soft robot with a simple curved configuration based on a PVDF unimorph film 119 , which can run fast with a relative speed of 20 body lengths per second in an oscillatory center of mass trajectory pattern Notably, it could stand the weight of an adult footstep because of the robustness provided by polymeric materials, showing the excellent compliance of these entirely flexible robots.
Soft magnetic artificial muscles
A magnetic field is effective in providing untethered driving force and control at the same time for microrobots 130 , 131 . Artificial muscles manipulated by magnetic fields are generally based on discrete or continuous magnetization profiles 13 . Individual micromagnets with proper soft joints are used for discrete magnetization profiles. The continuous magnetization profile requires magnetic particles dispersed across the entire soft polymer 7 , such as PDMS 132 or hydrogel 130 . These continuous magnetic composites tend to provide better compliance although the magnetization magnitudes are lower 13 .
As a significant advantage, soft magnetic artificial muscles are usually untethered. The magnetic field brings extra flexibility to this kind of artificial muscle, especially for in vivo operations in the human body. It can penetrate many materials and be spatially programmed 133 , 134 . An attractive application is microscopic artificial swimmers, which have been developing rapidly in recent years 130 , 135 , 136 , 137 . These microswimmers use a bioinspired flagellar propulsion mechanism with the construction composed of a microbody and flagellum. As shown in Fig. 7 a 130 , Huang et al. reported microswimmers based on a magnetic hydrogel and investigated the adaptive locomotion behavior of microswimmers in a complex fluidic and magnetic environment. In Fig. 7c , Ren et al. 138 . demonstrated a microswimmer that can selectively transport objects in water under the control of an external oscillating magnetic field. Magnetic composite elastomers (Ecoflex/NdFeB) were used as active driving components. Figure 7b presents a study on submillimeter-scale soft continuum robots based on ferromagnetic polymeric composites 132 . The hydrogel layer was used to cover the composites to reduce friction. Figure 7d shows the multilegged paddle crawling of a millimeter-scale soft robot in a microchannel filled with silicone oil. The robot was fabricated based on a newly developed UV lithography method for patterning permanent magnetic particles in a composite film 139 . A micromagnetic robot with a discrete magnetization profile is shown in Fig. 7 e 140 . Arrays of programable single-domain nanomagnets on connected panels can be operated in response to the applied magnetic field with high dexterity. The encoded magnetization direction is indicated with red arrows in the diagrams.
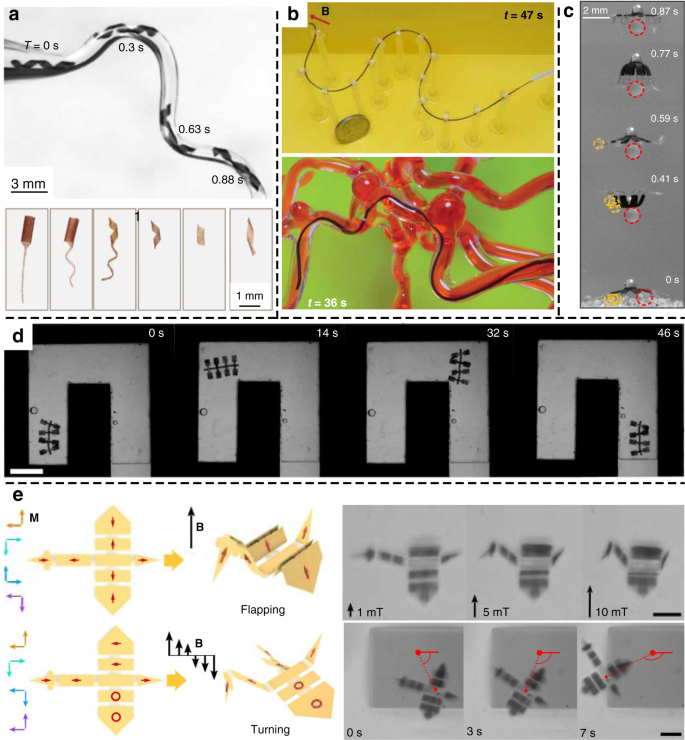
a Optical images of the engineered artificial microswimmers (upper) and motion of a flexible helix through a curved conduit (lower) 130 . Reproduced from ref. 130 (2019, CC BY 4.0). b Ferromagnetic soft continuum robots and navigation through a tortuous path (upper) and 3D cerebrovascular phantom network (lower) 132 . Copyright 2019 by AAAS. Reprinted with permission. c Micro jellyfish-like swimmer showing the capability of selective transportation of large beads 138 . Reproduced from ref. 138 (2019, CC BY 4.0). d A flexible robot with programmable three-dimensional magnetization and motion. (scale bar: 4 mm) 139 . Copyright 2019 by AAAS. Reprinted with permission. e Microscale origami bird with multiple shape-morphing modes (scale bars: 30 μm) 140 . Copyright 2019 by Springer Nature. Reprinted with permission
In addition to the above six categories of driving mechanisms, artificial muscles can be realized alternatively by molecular machines, chemical fuels (such as glucose 141 ), liquid crystal elastomers 142 , and thermoexpansion of polymers and nanocomposites 143 . For example, the development of artificial molecular machines suggests potential possibilities to mimic the natural macromolecular machines in muscles at the molecular level in the future, although current artificial molecular machines are often smaller and simpler than biological macromolecular machines. Particularly, the advent of a stimuli-responsive molecular machine developed by Livoreil et al. 144 and Bissell et al. 145 in 1994 has attracted much attention to this field. At the macroscale, some researchers have investigated artificial muscles powered by chemical reactions. Yang et al. developed autonomous crawling robots powered by the catalytic combustion of methanol 146 . Wehner et al. reported an entirely soft robot powered by the gas generated from catalytic decomposition of an onboard fuel 22 . In addition, He et al. reported a novel soft tubular actuator using an electrically controlled liquid crystal elastomer. This actuator provides multiple actuation modes, including contraction, bending, and expansion 142 . Recently, some microsurface electrochemical actuators 147 , 148 based on metal films have shown outstanding performance in aqueous environments, for instance, operating at ~1 V and achieving a submicrometer radius of curvature.
Stimulation-responsive gels are another promising option for artificial muscles. This field has developed very quickly in the past decade. Cohen Stuart et al. reviewed stimuli-responsive gels based on the self-assembly of nanoscale structures and materials 149 . Kouwer et al. presented thermoresponsive polyisocyanopeptide hydrogel networks that mimic cytoskeletal proteins 150 . Based on interpenetrating polymer networks, conductive responsive hydrogels have been developed to realize smart hydrogel artificial muscles with sensing functions 151 , 152 . Zhao et al. 152 demonstrated a soft somatosensitive actuator using an interpenetrating polymer double-network of poly(N- isopropylacrylamide) (PNIPAAm) and PANI.
Comparison of electrical and mechanical properties
The typical stress and strain characteristics of various artificial muscles are shown in Fig. 8 , with a skeletal muscle also included for reference. The circles provide the general ranges corresponding to specific mechanisms according to previous reviews 1 , 7 , 103 , 153 , 154 , 155 and some recent research. The data points show the maximum strain and stress ranges reported in the corresponding references: (a) SMA 1 , 156 , 157 and SMP 26 , 158 , 159 ; (b) pneumatic artificial muscles 156 , 160 ; (c) DEAs 1 , 82 , 83 , 161 , 162 , 163 ; (d) IPMCs 1 , 4 ; (e) piezoelectric artificial muscles 4 , 156 , 158 ; and (f) soft magnetic artificial muscles 7 , 164 , 165 . Among all the artificial muscles, the pneumatic artificial muscles cover the widest range of low-stress actuators. This is a combined effect of material selection, structural design, and power source. Soft magnet-polymer composites have a similar strain range to natural muscle, which suggests the potential to mimic the function and morphology of skeletal muscle. The SMPs can provide a high actuation force and work density with a relatively high strain. Although SMAs have the highest elastic modulus as materials, they are still applied in some soft actuators with low-stiffness structural design 41 .
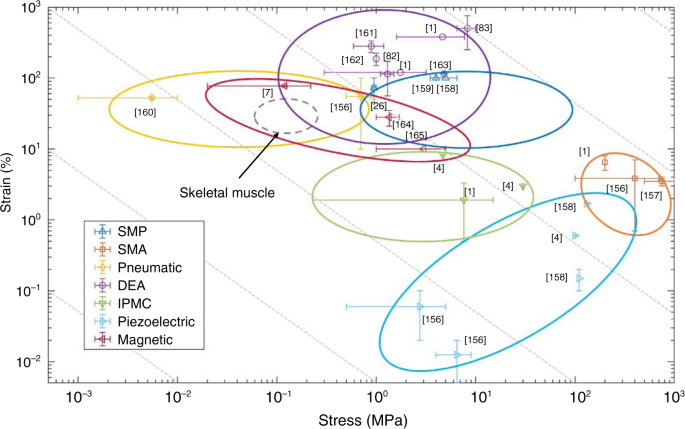
These artificial muscles include SMAs 1 , 156 , 157 and SMPs 26 , 158 , 159 , pneumatic artificial muscles 156 , 160 , DEAs 1 , 82 , 83 , 161 , 162 , 163 , IPMCs 1 , 4 , piezoelectric artificial muscles 4 , 156 , 158 , soft magnetic artificial muscles 7 , 164 , 165
Among the three kinds of EAPs, DE artificial muscles have the highest maximum strain levels, while IPMCs have a higher elastic modulus than DEs, which suggests that IPMCs can provide higher forces at the same actuation strain. Piezoelectric materials, especially piezoelectric ceramics such as PZT, have the highest stresses but lowest strains among EAPs. This makes piezoelectric actuators suitable for generating fine motion in structures of high mechanical impedance. For piezoelectric actuators, the proper motion amplifying method is required to achieve a larger motion range as discussed previously.
Table 1 presents a further comparison of the parameters of different mechanisms, including the range of maximum actuation strains, elastic moduli, work densities, efficiencies, and relative speeds 1 , 4 , 7 , 12 , 25 , 153 , 156 , 158 . Artificial muscles based on organic materials tend to have a high actuation strain and a low modulus, similar to natural skeletal muscle, while muscles containing metallic or ceramic components usually have a high elastic modulus. For thermal-responsive shape memory materials, the work densities are attractive; however, the issues of limited speed and low efficiency need to be explored and improved. Pneumatic soft actuators provide medium strain and speed similar to skeletal muscle, while the efficiency varies 23 , 160 , 166 . Electrical actuators such as DE and piezoelectric artificial muscles have high efficiency, which is significant for an autonomous system with a limited power supply. In addition, DEs and piezoelectric artificial muscles have high response speeds; therefore, they can be used in applications requiring high-frequency actuation, such as flapping-wing aerial robots 16 , 128 . The speed of IPMCs is relatively low, but their low operating voltage allows applications that cannot stand the high voltage required by the DE and piezoelectric artificial muscle. Soft magnetic composites have a medium strain level, work density and speed. The reported work density of a millimeter-scale actuator 167 was relatively low, on the order of 10 −2 kJ m −3 , which suggests that the work density of soft magnetic actuators could be limited at small scales. To date, only a limited number of studies have provided the efficiency of magnetic soft actuators. The spatial distribution of the magnetic field could lead to a relatively low efficiency; however, it allows the magnetic artificial muscles to be powered and controlled wirelessly.
Applications of artificial muscles
A large number of applications in various fields have been developed taking advantage of the diversity of artificial muscles. The selection of the actuation mechanism should comprehensively consider the material characteristics and actuation performance, such as the elastic modulus, strength, energy source, efficiency, and response time. Here, we will discuss the adaptability of certain artificial muscles to specific applications, according to the requirements of the application and the properties of the actuation mechanisms.
As shown in Table 2 , micro soft swimmers work in liquid environments such as water and blood and thus require extra waterproof properties, especially for electroactive materials. For untethered microswimmers working in the human body, magnetic actuation is preferred 130 , 137 , which allows wireless control and power transfer at the same time. Hydraulic actuators can be considered for microswimmers to potentially use the surrounding liquid, while it is challenging to develop micro pressure sources for untethered microswimmers. Low-voltage IPMCs with hydrogel materials may also work in water; however, the operating voltage should be below the decomposition voltage of the water.
Micro soft actuators for invasive surgery need the capability of fine motion, and the force should also be precisely controllable 168 , 169 . For microsurgery, soft artificial muscle can provide extra compliance and tolerance during invasive treatment. According to the required properties, the potential mechanisms include piezoelectric, magnetic, pneumatic and IPMC actuation. Piezoelectric 127 and magnetic 170 actuators can be manipulated with high precision and high speed. Pneumatic artificial muscles have excellent force and locomotion outputs and have be used in microsurgery 171 . However, the response time is relatively long, and the precaution of gas leakage should be considered in some microsurgeries. In addition, IPMCs with low operating voltages provide a safe option, but it is still challenging for an IPMC to provide fine motion compared to a piezoelectric actuator.
Micro flying robots usually need high-frequency actuation up to several hundred hertz, which is challenging for many artificial muscles. To drive the wings of micro flying robots, piezoelectric materials 17 and DEAs 16 have been used because of the short response time and acceptable produced force. In addition, micro SMA actuators can potentially be used in this application considering that they have shown the ability to operate above 1 kHz 44 , and the produced force is relatively high. As most SMAs show a one-way shape memory effect, proper structural design is needed to provide periodic movement.
Micropumps can be used in microfluidic devices. The stroke should be large to maximize the driving ability, while the response time should be low to achieve fast control of fluidic flows. A waterproof artificial muscle is suggested considering robustness. Pneumatic actuators 172 can generate large strain and relatively high force, while piezoelectric actuators 107 can provide fine control for precise micropumps. IPMCs are able to generate relatively large strokes, and a low operation voltage increases safety 173 , 174 . Magnetic micropumps are promising with flexible wireless power transmission and control; however, the produced force and power might decline rapidly at the microscale considering the scaling law 13 .
As discussed in previous sections, many mechanisms can be used in soft grippers; here, we provide some examples. Although the requirement for soft grippers varies according to the specific properties of objects and the ambient environment, such as the hardness of materials, chemical components, and ambient temperature, the most basic and general requirement of soft grippers as actuators is the fine control of force and stroke. Pneumatic artificial muscles are most widely explored for this purpose, and many studies have demonstrated their outstanding performance. In addition, soft IPMCs can provide a large stroke using low voltage, and film-based structures of IPMCs need less space in microsystems than pneumatic artificial muscle. However, the relatively low precision and low response speed are considered to be limitations for the application of IPMCs.
Artificial insect exoskeletons can provide the driving force for insect-scale robots, which require actuators with a high energy density, a large stroke and a quick response. Piezoelectric polymers 119 , 120 and IPMCs 98 have been shown to be suitable for this application. An additional advantage of piezoelectric artificial muscles is the high energy conversion efficiency, which allows a longer lifetime for autonomous robotic insects powered by batteries. Micropneumatic actuators are promising and will benefit from studies on pneumatic mechanisms for macroscale robots. The main challenge is the miniaturization of efficient pumps. DEs can provide powerful actuation and a quick response for robotic insects, although the high operating voltage requires relatively complex onboard circuitry 175 .
In addition, scaling laws of various artificial muscles should be considered for designing small-scale devices. Generally, the actuation force and energy are dependent on a characteristic length 176 , 177 . Diller et al. provided a detailed analysis on this issue 176 . When the scale is below 1 µm, the surface area-to-volume ratio of a microactuator becomes high, and surface-area effects are critical. Viscous forces, friction, and adhesion should not be neglected, and they can be utilized to drive microactuators 12 .
Energy delivery, storage, and conversion in microsystems
The basic function of the actuation microsystem is to convert energy in various forms into mechanical energy with the required force and motion. Therefore, it is worth considering the most efficient energy management strategies (including energy delivery, storage, and conversion) in this context. Pursuing autonomy is particularly important and challenging for microsystems. Soft robots are typical autonomous systems actuated by artificial muscles. According to Rus and Tolley’s definition, a basic property of soft robots as systems is their capability of autonomous behavior 66 . For autonomous microsystems, untethered energy delivery, with or without local storage, is preferred. In this section, we will focus on energy issues, mainly discussing opportunities in developing energy-autonomous microrobot systems by introducing multistage distributed energy and actuation management.
A paradigm of autonomous microsystems with energy and actuation management can be summarized as shown in Fig. 9 . The energy management strategy includes energy storage and delivery, while actuation management involves centralized actuation generation and delivery. The block diagram of Fig. 9 can be thought of as a tool box containing elements that can be repeated and combined in different architectures according to system needs. In the following paragraphs, we will discuss relevant details of these two aspects.
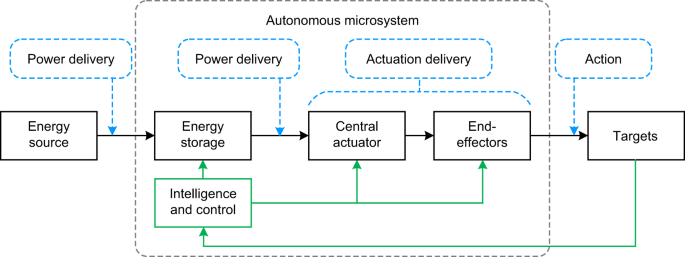
Untethered power supplies and proper local energy storage can help microsystems to realize long-term energy autonomy. For microsystems with multiple end-effectors, centralized actuation has the potential to improve the actuating capability and efficiency while minimizing the structural complexity and fabrication difficulty

Energy delivery and storage
A biological example of energy delivery and storage in an autonomous system is the human digestive system and circulatory system. Muscles in the human body consume energy all the time, but people only need to eat food two or three times per day or less to fulfill the daily energy requirement. The energy in the food is taken into the human body intensively in a short time, after which the body uses several stages of energy storage and conversion as “buffers” to make the energy released to tissues slow and smooth. The existence of these energy buffers, such as stomach, liver, and adipose tissue, gives people extraordinary freedom to work and play rather than taking all the time to eat. The above mechanism realizes energy delivery from the environment to the human body and energy storage in the body. Thereafter, the blood in the circulatory system carries nutrients and other essential substances (such as glucose and oxygen) throughout the body. For muscle cells, the blood provides both nourishment and energy, such as glucose, by flowing through the vessels throughout the body to maintain their basic functions and enable their movement under the control of the nervous system. Local chemical energy storage in muscle allows rapid conversion to mechanical effort when the higher output power is needed than can be supplied by the energy delivery rate of the blood.
This biological mechanism is inspiring for the design of autonomous robotic systems. The power and control signals can be delivered in a tethered fashion to the robot body; however, this tethered connection limits the robot’s flexibility. This is especially problematic when the behavior of the robot is complex (such as flying) or when the number of robots becomes large (such as robot swarms). In addition, the low power consumption of the microactuator allows us to choose untethered power supplies with limited power density. Therefore, proper local energy storage and management, as shown in Fig. 9 , can coordinate the energy release on demand for actuators in the system temporally and spatially. Rechargeable batteries are the most commonly used energy storage devices for general purposes, while supercapacitors are well suited for applications requiring high power for low duration. By combining one or more of these storage devices with existing wireless power transmission technology 178 , 179 , 180 , the microrobot system can realize long-term energy autonomy. Less explored to date is the wide range of options for centralized, distributed, or localized energy storage. As robotic microsystems become more complex, this design space can be explored to maximize performance within the constraints of space, operating environment, and functional requirements.
In addition to active power delivery, energy harvesting can help by converting ambient energy 181 , 182 into electrical power, such as solar cells 183 , 184 , vibration energy harvesters 185 , stretchable rectennas for RF energy harvesting 186 , biofuel cells 187 , stretchable triboelectric and piezoelectric nanogenerators 114 , 188 , 189 . Over the past two decades, major advances in energy harvesting have allowed the emergence of various energy-autonomous devices powered. Micro energy harvesters can be integrated into low-power microactuators or systems. Local storage provision is usually essential in such systems to provide the required energy buffer when demand does not coincide with the availability of supply. In this way, microsystems have opportunities to be entirely energy-autonomous in the future.
Centralized actuation and actuation delivery
In many scenarios, such as invasive robotic surgery, we can find that several collaborative end effectors could be necessary to complete a task. Conventionally, in these cases, independent driving components are assigned for each end effector. For macroscale or mesoscale robots and actuators, this architecture provides good flexibility for manipulation. However, it causes extra difficulties when the system is scaled down to the microscale. If each of the microactuators requires independent power and actuation arrangements, then the complexity of system design and fabrication will significantly increase.
A promising alternative architecture is centralized multistage actuation. In this architecture, the actuation required by end effectors can be generated by a single high-power central transducer, as shown in Fig. 9 . The actuation can be delivered to each end effector in the next stage with a proper transmission mechanism (such as hydraulic, pneumatic, or mechanical linkages such as tendons), so no extra actuating transducer needs to be embedded in these end effectors. Benefiting from centralized actuation, the scale, structural complexity, and fabrication difficulty can be minimized. Moreover, because the central actuator can be placed out of the narrow workspace, it can be designed with more freedom. Compared to the limited scale for an actuator on the end effector, centralized actuation has the potential to improve the maximum actuating capability according to the specific requirement, thus enhancing the performance of the microsystem. Here, macroscale robotic systems also provide inspiration. In macroscale systems, centralized transduction also allows the use of complex, high-efficiency devices (such as hydraulic pumps) while avoiding size constraints and costs or multiple local transducers.
For example, multi-degree-of-freedom microactuators in microsurgery are usually driven by several local actuators; each actuator needs power and control. This makes it hard to decrease the scale of the microactuators. In addition, it can be unsafe when considering the high driving voltage of piezoelectric actuators or the risk of gas leakage of pneumatic actuators. In this case, a central actuator can be used to generate actuation, while a suitable actuation transmission mechanism (such as a hydraulic or leveraged system) can be used to adjust and transfer the required actuation to single or multiple probes at the end. For this architecture, major limitations include material strength and design of the actuation transmission mechanisms, as well as speed limitations and artifacts such as backlash.
Conclusions
Studies of artificial muscle represent a rapidly growing field. These studies provide innovative approaches for generating actuation with soft materials of outstanding compliance. Here, we have discussed the recent progress in artificial muscle research involving different driving mechanisms, including shape memory materials, pneumatic and hydraulic microactuators, DEs, ionic artificial muscles, piezoelectric microactuators, and micromagnetic artificial muscles.
Each mechanism has pros and cons, so it is suited for different applications. We have discussed preferred driving mechanisms for some typical applications. Output power levels of artificial muscles have been improving in the past decade. Some advanced artificial muscles have already shown competitive power density compared to natural skeletal muscle, but the efficiency of most mechanisms remains at a low level except for the piezoelectric mechanism. The long response time due to the viscoelasticity of polymers limits their use in applications requiring high-frequency movement. Although some studies have been conducted to solve this problem, it is still challenging especially for SMPs and ionic artificial muscles.
We introduce a multistage energy and actuation management paradigm for energy-autonomous microsystems. Flexible energy delivery and local energy storage are promising for improving the reliability and quality of the energy supply and can contribute to the energy autonomy of the microsystem. Centralized actuation generation can potentially improve the overall power efficiency and simplify the design of end effectors in microsystems. Several actuation delivery strategies may be considered for delivering actuation between the central actuator and end effectors. Despite its exploratory nature, this study offers some insight into energy and actuation management for future research and practice.
Artificial muscles are competitive for replacing conventional actuators in areas requiring strong human-machine interaction and strong adaptability to the surrounding environment. In the future, it will be attractive to use this technology for in vivo testing and treatment, drug delivery, micromanipulation of biomaterials, and assistance with physical disabilities. By combining artificial muscle with rapidly developing electronic skin, artificial intelligence algorithms, and wireless power transfer, it is promising for realizing autonomy in many inspiring tasks, such as rehabilitation assistance and swarm intelligence of microrobots in complex environments.
Madden, J. D. W. et al. Artificial muscle technology: physical principles and naval prospects. IEEE J. Ocean. Eng. 29 , 706–728 (2004).
Article Google Scholar
Barrett, K. E., Barman, S. M., Brooks, H. L. & Yuan, J. X.-J. Excitable Tissue: Muscle. in Ganong’s Review of Medical Physiology , 26e (McGraw-Hill Education LLC., 2019).
Whitesides, G. M. Soft robotics. Angew. Chem. Int. Ed. 57 , 4258–4273 (2018).
Mirvakili, S. M. & Hunter, I. W. Artificial muscles: mechanisms, applications, and challenges. Adv. Mater. 30 , 1704407 (2018).
Duduta, M., Hajiesmaili, E., Zhao, H., Wood, R. J. & Clarke, D. R. Realizing the potential of dielectric elastomer artificial muscles. Proc. Natl Acad. Sci. USA 116 , 2476–2481 (2019).
Haines, C. S. et al. Artificial muscles from fishing line and sewing thread. Science 343 , 868–872 (2014).
Nguyen, V. Q., Ahmed, A. S. & Ramanujan, R. V. Morphing soft magnetic composites. Adv. Mater. 24 , 4041–4054 (2012).
Majidi, C. Soft‐matter engineering for soft robotics. Adv. Mater. Technol. 4 , 1800477 (2018).
Qiu, Y., Zhang, E., Plamthottam, R. & Pei, Q. Dielectric elastomer artificial muscle: materials innovations and device explorations. Acc. Chem. Res. 52 , 316–325 (2019).
De Luca, V. et al. Ionic electroactive polymer metal composites: fabricating, modeling, and applications of postsilicon smart devices. J. Polym. Sci. Part B Polym. Phys. 51 , 699–734 (2013).
Gupta, V., Sharma, M. & Thakur, N. Optimization criteria for optimal placement of piezoelectric sensors and actuators on a smart structure: a technical review. J. Intell. Mater. Syst. Struct. 21 , 1227–1243 (2010).
Behl, M., Razzaq, M. Y. & Lendlein, A. Multifunctional shape-memory polymers. Adv. Mater. 22 , 3388–3410 (2010).
Hines, L., Petersen, K., Lum, G. Z. & Sitti, M. Soft actuators for small-scale robotics. Adv. Mater. 29 , 1603483 (2017).
Mirvakili, S. M. & Hunter, I. W. Multidirectional artificial muscles from nylon. Adv. Mater. 29 , 1–7 (2017).
Pelrine, R., Kornbluh, R., Pei, Q. & Joseph, J. High-speed electrically actuated elastomers with strain greater than 100%. Science 287 , 836–839 (2000).
Chen, Y. et al. Controlled flight of a microrobot powered by soft artificial muscles. Nature 575 , 324–329 (2019).
Ma, K. Y., Chirarattananon, P., Fuller, S. B. & Wood, R. J. Controlled flight of a biologically inspired, insect-scale robot. Science 340 , 603–607 (2013).
Lee, D. W. et al. Magnetic torsional actuation of carbon nanotube yarn artificial muscle. RSC Adv. 8 , 17421–17425 (2018).
Jeon, S. et al. A magnetically controlled soft microrobot steering a guidewire in a three-dimensional phantom vascular network. Soft Robot. 6 , 54–68 (2019).
Yang, L. et al. A powerful dual-responsive soft actuator and photo-to-electric generator based on graphene micro-gasbags for bioinspired applications. J. Mater. Chem. B 6 , 5031–5038 (2018).
Lima, M. D. et al. Electrically, chemically, and photonically powered torsional and tensile actuation of hybrid carbon nanotube yarn muscles. Science 338 , 928–932 (2012).
Wehner, M. et al. An integrated design and fabrication strategy for entirely soft, autonomous robots. Nature 536 , 451–455 (2016).
Ainla, A., Verma, M. S., Yang, D. & Whitesides, G. M. Soft, rotating pneumatic actuator. Soft Robot. 4 , 297–304 (2017).
Mu, J. et al. Sheath-run artificial muscles. Science 365 , 150–155 (2019).
Lendlein, A. & Gould, O. E. C. Reprogrammable recovery and actuation behaviour of shape-memory polymers. Nat. Rev. Mater. 4 , 116–133 (2019).
Xie, T. Tunable polymer multi-shape memory effect. Nature 464 , 267–270 (2010).
Mohd Jani, J., Leary, M., Subic, A. & Gibson, M. A. A review of shape memory alloy research, applications and opportunities. Mater. Des. 56 , 1078–1113 (2014).
Song, Y., Chen, X., Dabade, V., Shield, T. W. & James, R. D. Enhanced reversibility and unusual microstructure of a phase-transforming material. Nature 502 , 85–88 (2013).
Swain, M. V. Shape memory behaviour in partially stabilized zirconia ceramics. Nature 322 , 234–236 (1986).
Lai, A., Du, Z., Gan, C. L. & Schuh, C. A. Shape memory and superelastic ceramics at small scales. Science 341 , 1505–1508 (2013).
Lendlein, A. & Kelch, S. Shape-memory polymers. Angew. Chem. Int. Ed. 41 , 2034 (2002).
Meng, H. & Li, G. A review of stimuli-responsive shape memory polymer composites. Polymer 54 , 2199–2221 (2013).
Ölander, A. A. N. Electrochemical investigation of solid cadmium-gold alloys. J. Am. Chem. Soc. 54 , 3819–3833 (1932).
Kauffman, G. B. & Mayo, I. The story of nitinol: the serendipitous discovery of the memory metal and its applications. Chem. Educ. 2 , 1–21 (1997).
Sun, L. & Huang, W. M. Nature of the multistage transformation in shape memory alloys upon heating. Met. Sci. Heat. Treat. 51 , 573–578 (2009).
An, L., Huang, W. M., Fu, Y. Q. & Guo, N. Q. A note on size effect in actuating NiTi shape memory alloys by electrical current. Mater. Des. 29 , 1432–1437 (2008).
Yu, L. et al. Multi-shape-memory effects in a wavelength-selective multicomposite. J. Mater. Chem. A 3 , 13953–13961 (2015).
Luo, X. & Mather, P. T. Triple-shape polymeric composites (TSPCs). Adv. Funct. Mater. 20 , 2649–2656 (2010).
Behl, M., Kratz, K., Zotzmann, J., Nöchel, U. & Lendlein, A. Reversible bidirectional shape-memory polymers. Adv. Mater. 25 , 4466–4469 (2013).
Lendlein, A. Fabrication of reprogrammable shape-memory polymer actuators for robotics. Sci. Robot. 3 , 2–4 (2018).
Huang, X. et al. Chasing biomimetic locomotion speeds: creating untethered soft robots with shape memory alloy actuators. Sci. Robot. 3 , eaau7557 (2018).
Xiao, R. & Huang, W. M. Heating/solvent responsive shape‐memory polymers for implant biomedical devices in minimally invasive surgery: current status and challenge. Macromol. Biosci. 20 , 2000108 (2020).
Kim, S. et al. Micro artificial muscle fiber using NiTi spring for soft robotics. in 2009 IEEE/RSJ International Conference on Intelligent Robots and Systems 2228–2234 (IEEE, 2009). https://doi.org/10.1109/IROS.2009.5354178 .
Lee, H.-T., Kim, M.-S., Lee, G.-Y., Kim, C.-S. & Ahn, S.-H. Shape memory alloy (SMA)-based microscale actuators with 60% deformation rate and 1.6 kHz actuation speed. Small 14 , 1801023 (2018).
Xu, H. et al. Deformable, programmable, and shape- memorizing micro-optics. Adv. Funct. Mater. 23 , 3299–3306 (2013).
Zhang, W. et al. Structural multi-colour invisible inks with submicron 4D printing of shape memory polymers. Nat. Commun. 12 , 112 (2021).
Felton, S. M. et al. Self-folding with shape memory composites. Soft Matter 9 , 7688–7694 (2013).
Zhao, Q., Zou, W., Luo, Y. & Xie, T. Shape memory polymer network with thermally distinct elasticity and plasticity. Sci. Adv. 2 , e1501297 (2016).
Zhang, Y. et al. Climbing-inspired twining electrodes using shape memory for peripheral nerve stimulation and recording. Sci. Adv. 5 , eaaw1066 https://doi.org/10.1126/sciadv.aaw1066 (2019).
Jin, B. et al. Programming a crystalline shape memory polymer network with thermo- and photo-reversible bonds toward a single-component soft robot. Sci. Adv. 4 , eaao3865 (2018).
Zarek, M. et al. 3D printing of shape memory polymers for flexible electronic devices. Adv. Mater. 28 , 4449–4454 (2016).
Zhang, Y. et al. 4D printing of a digital shape memory polymer with tunable high performance. ACS Appl. Mater. Interfaces 11 , 32408–32413 (2019).
Ze, Q. et al. Magnetic shape memory polymers with integrated multifunctional shape manipulation. Adv. Mater. 32 , 1–8 (2020).
Google Scholar
Gurevitch, I. & Silverstein, M. S. Shape memory polymer foams from emulsion templating. Soft Matter 8 , 10378 (2012).
Wei, K., Zhu, G., Tang, Y. & Li, X. Electroactive shape-memory effects of hydro-epoxy/carbon black composites. Polym. J. 45 , 671–675 (2013).
Heidarshenas, M., Kokabi, M. & Hosseini, H. Shape memory conductive electrospun PVA/MWCNT nanocomposite aerogels. Polym. J. 51 , 579–590 (2019).
Yu, K., Liu, Y. & Leng, J. Shape memory polymer/CNT composites and their microwave induced shape memory behaviors. RSC Adv. 4 , 2961–2968 (2014).
Yuan, J. et al. Shape memory nanocomposite fibers for untethered high-energy microengines. Science 365 , 155–158 (2019).
Gorissen, B., Vincentie, W., Al-Bender, F., Reynaerts, D. & De Volder, M. Modeling and bonding-free fabrication of flexible fluidic microactuators with a bending motion. J. Micromech. Microeng. 23 , 045012 (2013).
Paek, J., Cho, I. & Kim, J. Microrobotic tentacles with spiral bending capability based on shape-engineered elastomeric microtubes. Sci. Rep. 5 , 10768 (2015).
Skylar-Scott, M. A., Mueller, J., Visser, C. W. & Lewis, J. A. Voxelated soft matter via multimaterial multinozzle 3D printing. Nature 575 , 330–335 (2019).
Ge, L., Dong, L., Wang, D., Ge, Q. & Gu, G. A digital light processing 3D printer for fast and high-precision fabrication of soft pneumatic actuators. Sens. Actuators A Phys. 273 , 285–292 (2018).
Amiri Moghadam, A. A. et al. Laser cutting as a rapid method for fabricating thin soft pneumatic actuators and robots. Soft Robot. 5 , 443–451 (2018).
Gorissen, B., Van Hoof, C., Reynaerts, D. & De Volder, M. SU8 etch mask for patterning PDMS and its application to flexible fluidic microactuators. Microsyst. Nanoeng. 2 , 16045 (2016).
De Volder, M. & Reynaerts, D. Pneumatic and hydraulic microactuators: a review. J. Micromech. Microeng. 20 , 043001 (2010).
Rus, D. & Tolley, M. T. Design, fabrication and control of soft robots. Nature 521 , 467–475 (2015).
Wehner, M. et al. Pneumatic energy sources for autonomous and wearable soft robotics. Soft Robot. 1 , 263–274 (2014).
Pang, C., Tai, Y.-C., Burdick, J. W. & Andersen, R. Electrolysis-based diaphragm actuators. Nanotechnology 17 , S64–S68 (2006).
Gorissen, B. et al. Flexible pneumatic twisting actuators and their application to tilting micromirrors. Sens. Actuators A Phys. 216 , 426–431 (2014).
Hawkes, E. W., Blumenschein, L. H., Greer, J. D. & Okamura, A. M. A soft robot that navigates its environment through growth. Sci. Robot. 2 , eaan3028 (2017).
Blumenschein, L. H., Gan, L. T., Fan, J. A., Okamura, A. M. & Hawkes, E. W. A tip-extending soft robot enables reconfigurable and deployable antennas. IEEE Robot. Autom. Lett. 3 , 949–956 (2018).
Mirvakili, S. M., Sim, D., Hunter, I. W. & Langer, R. Actuation of untethered pneumatic artificial muscles and soft robots using magnetically induced liquid-to-gas phase transitions. Sci. Robot. 5 , eaaz4239 (2020).
Song, S. & Sitti, M. Soft grippers using micro- fibrillar adhesives for transfer printing. Adv. Mater. 26 , 4901–4906 (2014).
Konishi, S., Shimomura, S., Tajima, S. & Tabata, Y. Implementation of soft microfingers for a hMSC aggregate manipulation system. Microsyst. Nanoeng. 2 , 15048 (2016).
Diteesawat, R. S., Helps, T., Taghavi, M. & Rossiter, J. Electro-pneumatic pumps for soft robotics. Sci. Robot. 6 , eabc3721 (2021).
Brochu, P. & Pei, Q. Advances in dielectric elastomers for actuators and artificial muscles. Macromol. Rapid Commun. 31 , 10–36 (2010).
Anderson, I. A., Gisby, T. A., McKay, T. G., O’Brien, B. M. & Calius, E. P. Multi-functional dielectric elastomer artificial muscles for soft and smart machines. J. Appl. Phys. 112 , 041101 (2012).
Plante, J.-S. & Dubowsky, S. On the properties of dielectric elastomer actuators and their design implications. Smart Mater. Struct. 16 , S227–S236 (2007).
Zhang, H. et al. Interpenetrating polymer networks based on acrylic elastomers and plasticizers with improved actuation temperature range. Polym. Int. 59 , 384–390 (2010).
Carpi, F. & De Rossi, D. Improvement of electromechanical actuating performances of a silicone dielectric elastomer by dispersion of titanium dioxide powder. IEEE Trans. Dielectr. Electr. Insul. 12 , 835–843 (2005).
Hu, W., Zhang, S. N., Niu, X., Liu, C. & Pei, Q. An aluminum nanoparticle–acrylate copolymer nanocomposite as a dielectric elastomer with a high dielectric constant. J. Mater. Chem. C 2 , 1658 (2014).
Molberg, M. et al. High breakdown field dielectric elastomer actuators using encapsulated polyaniline as high dielectric constant filler. Adv. Funct. Mater. 20 , 3280–3291 (2010).
Li, C.-H. et al. A highly stretchable autonomous self- healing elastomer. Nat. Chem. 8 , 618–624 (2016).
Madsen, F. B., Yu, L. & Skov, A. L. Self-healing, high-permittivity silicone dielectric elastomer. ACS Macro Lett. 5 , 1196–1200 (2016).
Duan, L., Lai, J. C., Li, C. H. & Zuo, J. L. A dielectric elastomer actuator that can self-heal integrally. ACS Appl. Mater. Interfaces 12 , 44137–44146 (2020).
Ji, X. et al. An autonomous untethered fast soft robotic insect driven by low-voltage dielectric elastomer actuators. Sci. Robot. 4 , eaaz6451 (2019).
Shahinpoor, M. & Kim, K. J. Ionic polymer-metal composites: I. Fundamentals. Smart Mater. Struct. 10 , 819–833 (2001).
Bahramzadeh, Y. & Shahinpoor, M. A review of ionic polymeric soft actuators and sensors. Soft Robot. 1 , 38–52 (2014).
Kong, L. & Chen, W. Carbon nanotube and graphene-based bioinspired electrochemical actuators. Adv. Mater. 26 , 1025–1043 (2014).
Lu, L. & Chen, W. Biocompatible composite actuator: a supramolecular structure consisting of the biopolymer chitosan, carbon nanotubes, and an ionic liquid. Adv. Mater. 22 , 3745–3748 (2010).
Lu, L. et al. Highly stable air working bimorph actuator based on a graphene nanosheet/carbon nanotube hybrid electrode. Adv. Mater. 24 , 4317–4321 (2012).
Maziz, A. et al. Knitting and weaving artificial muscles. Sci. Adv. 3 , 1–12 (2017).
Kim, O., Kim, H., Choi, U. H. & Park, M. J. One-volt- driven superfast polymer actuators based on single-ion conductors. Nat. Commun. 7 , 13576 (2016).
Umrao, S. et al. MXene artificial muscles based on ionically cross-linked Ti3C2Tx electrode for kinetic soft robotics. Sci. Robot. 4 , 1–12 (2019).
Lu, C. et al. High-performance graphdiyne-based electrochemical actuators. Nat. Commun. 9 , 1–11 (2018).
Ma, S. et al. High‐performance ionic‐polymer–metal composite: toward large‐deformation fast‐ response artificial muscles. Adv. Funct. Mater. 30 , 1908508 (2020).
Must, I. et al. Ionic and capacitive artificial muscle for biomimetic soft robotics. Adv. Eng. Mater. 17 , 84–94 (2015).
Chen, Z. A review on robotic fish enabled by ionic polymer–metal composite artificial muscles. Robot. Biomim. 4 , 24 (2017).
Zhang, Y. S. & Khademhosseini, A. Advances in engineering hydrogels. Science 356 , eaaf3627 (2017).
Liu, X., Liu, J., Lin, S. & Zhao, X. Hydrogel machines. Mater. Today 36 , 102–124 (2020).
Yang, C. & Suo, Z. Hydrogel ionotronics. Nat. Rev. Mater. 3 , 125–142 (2018).
Mirfakhrai, T., Madden, J. D. W. & Baughman, R. H. Polymer artificial muscles. Mater. Today 10 , 30–38 (2007).
Michael, A. & Kwok, C. Y. Piezoelectric micro-lens actuator. Sens. Actuators A Phys. 236 , 116–129 (2015).
Xu, W. & Wu, Y. Piezoelectric actuator for machining on macro-to-micro cylindrical components by a precision rotary motion control. Mech. Syst. Signal Process. 114 , 439–447 (2019).
Chen, W. et al. Design and experimental evaluation of a novel stepping linear piezoelectric actuator. Sens. Actuators A Phys. 276 , 259–266 (2018).
Nguyen, N.-T. & Truong, T.-Q. A fully polymeric micropump with piezoelectric actuator. Sens. Actuators B Chem. 97 , 137–143 (2004).
Jain, R. K., Majumder, S., Ghosh, B. & Saha, S. Design and manufacturing of mobile micro manipulation system with a compliant piezoelectric actuator based micro gripper. J. Manuf. Syst. 35 , 76–91 (2015).
DONG, S. Review on piezoelectric, ultrasonic, and magnetoelectric actuators. J. Adv. Dielectr. 02 , 1230001 (2012).
Gao, X. et al. Piezoelectric actuators and motors: materials, designs, and applications. Adv. Mater. Technol. 5 , 1–26 (2020).
Cui, H. et al. Three-dimensional printing of piezoelectric materials with designed anisotropy and directional response. Nat. Mater. 18 , 234–241 (2019).
Guerin, S., Tofail, S. A. M. & Thompson, D. Organic piezoelectric materials: milestones and potential. NPG Asia Mater. 11 , 10 (2019).
Zhang, Y., Peng, Y., Sun, Z. & Yu, H. A novel stick–slip piezoelectric actuator based on a triangular compliant driving mechanism. IEEE Trans. Ind. Electron. 66 , 5374–5382 (2019).
Yang, Z., Zhou, S., Zu, J. & Inman, D. High-performance piezoelectric energy harvesters and their applications. Joule 2 , 642–697 (2018).
Safaei, M., Sodano, H. A. & Anton, S. R. A review of energy harvesting using piezoelectric materials: state-of-the-art a decade later (2008–2018). Smart Mater. Struct. 28 , 113001 (2019).
Villa, S. M. et al. Soft piezoionic/piezoelectric nanocomposites based on ionogel/BaTiO3 nanoparticles for low frequency and directional discriminative pressure sensing. ACS Macro Lett. 8 , 414–420 (2019).
Khanbareh, H. et al. Large area and flexible micro-porous piezoelectric materials for soft robotic skin. Sens. Actuators A Phys. 263 , 554–562 (2017).
Shi, M., Holmes, A. S. & Yeatman, E. M. Piezoelectric wind velocity sensor based on the variation of galloping frequency with drag force. Appl. Phys. Lett. 116 , 264101 (2020).
Wu, Y. et al. Insect-scale fast moving and ultrarobust soft robot. Sci. Robot. 4 , aax1594 https://doi.org/10.1126/scirobotics.aax1594 (2019).
Park, T. & Cha, Y. Soft mobile robot inspired by animal-like running motion. Sci. Rep. 9 , 14700 (2019).
Liu, Y. Z. et al. A high-performance soft actuator based on a poly(vinylidene fluoride) piezoelectric bimorph. Smart Mater. Struct. 28 , 055011 (2019).
Conway, N. J., Traina, Z. J. & Kim, S.-G. A strain amplifying piezoelectric MEMS actuator. J. Micromech. Microeng. 17 , 781–787 (2007).
Vanderborght, B. et al. Variable impedance actuators: a review. Rob. Auton. Syst. 61 , 1601–1614 (2013).
Nayak, S. & Rao, M. Design and development of a flexurally amplified piezoelectric actuator based piezo-hydraulic pump. Mater. Today Proc. https://doi.org/10.1016/j.matpr.2021.03.314 (2021).
Kiziroglou, M. E., Temelkuran, B., Yeatman, E. M. & Yang, G. Z. Micro motion amplification—a review. IEEE Access 8 , 64037–64055 (2020).
McClintock, H., Temel, F. Z., Doshi, N., Koh, J. & Wood, R. J. The milliDelta: a high-bandwidth, high-precision, millimeter-scale Delta robot. Sci. Robot. 3 , eaar3018 (2018).
Suzuki, H. & Wood, R. J. Origami-inspired miniature manipulator for teleoperated microsurgery. Nat. Mach. Intell. 2 , 437–446 (2020).
Jafferis, N. T., Helbling, E. F., Karpelson, M. & Wood, R. J. Untethered flight of an insect-sized flapping-wing microscale aerial vehicle. Nature 570 , 491–495 (2019).
Goldberg, B. et al. Power and control autonomy for high-speed locomotion with an insect-scale legged robot. IEEE Robot. Autom. Lett. 3 , 987–993 (2018).
Huang, H.-W. et al. Adaptive locomotion of artificial microswimmers. Sci. Adv. 5 , eaau1532 (2019).
Alapan, Y., Bozuyuk, U., Erkoc, P., Karacakol, A. C. & Sitti, M. Multifunctional surface microrollers for targeted cargo delivery in physiological blood flow. Sci. Robot. 5 , eaba5726 (2020).
Kim, Y., Parada, G. A., Liu, S. & Zhao, X. Ferromagnetic soft continuum robots. Sci. Robot. 4 , eaax7329 (2019).
Wang, J., Gao, D. & Lee, P. S. Recent progress in artificial muscles for interactive soft robotics. Adv. Mater. https://doi.org/10.1002/adma.202003088 (2020).
Miao, L. et al. 3D temporary‐magnetized soft robotic structures for enhanced energy harvesting. Adv. Mater. https://doi.org/10.1002/adma.202102691 (2021).
Dreyfus, R. et al. Microscopic artificial swimmers. Nature 437 , 862–865 (2005).
Jang, B. et al. Undulatory locomotion of magnetic multilink nanoswimmers. Nano Lett. 15 , 4829–4833 (2015).
Huang, H. W., Sakar, M. S., Petruska, A. J., Pané, S. & Nelson, B. J. Soft micromachines with programmable motility and morphology. Nat. Commun. 7 , 1–10 (2016).
Ren, Z., Hu, W., Dong, X. & Sitti, M. Multi- functional soft-bodied jellyfish-like swimming. Nat. Commun. 10, 2703 https://doi.org/10.1038/s41467-019-10549-7 (2019).
Xu, T., Zhang, J., Salehizadeh, M., Onaizah, O. & Diller, E. Millimeter-scale flexible robots with programmable three-dimensional magnetization and motions. Sci. Robot. 4 , eaav4494 (2019).
Cui, J. et al. Nanomagnetic encoding of shape-morphing micromachines. Nature 575 , 164–168 (2019).
Mashayekhi Mazar, F. et al. Artificial muscles powered by glucose. Adv. Mater. 31 , 1901677 (2019).
He, Q. et al. Electrically controlled liquid crystal elastomer–based soft tubular actuator with multimodal actuation. Sci. Adv. 5 , eaax5746 (2019).
Hu, Y. et al. Electrically and sunlight-driven actuator with versatile biomimetic motions based on rolled carbon nanotube bilayer composite. Adv. Funct. Mater. 27 , 1704388 (2017).
Livoreil, A., Dietrich-Buchecker, C. O. & Sauvage, J.-P. Electrochemically triggered swinging of a [2]-catenate. J. Am. Chem. Soc. 116 , 9399–9400 (1994).
Bissell, R. A., Córdova, E., Kaifer, A. E. & Stoddart, J. F. A chemically and electrochemically switchable molecular shuttle. Nature 369 , 133–137 (1994).
Yang, X., Chang, L. & Pérez-Arancibia, N. O. An 88-milligram insect-scale autonomous crawling robot driven by a catalytic artificial muscle. Sci. Robot. 5 , 1–14 (2020).
Miskin, M. Z. et al. Electronically integrated, mass-manufactured, microscopic robots. Nature 584 , 557–561 (2020).
Liu, Q. et al. Micrometer-sized electrically programmable shape-memory actuators for low-power microrobotics. Sci. Robot. 6 , eabe6663 (2021).
Stuart, Ma. C. et al. Emerging applications of stimuli-responsive polymer materials. Nat. Mater. 9 , 101–113 (2010).
Kouwer, P. H. J. et al. Responsive biomimetic networks from polyisocyanopeptide hydrogels. Nature 493 , 651–655 (2013).
Shi, Y., Ma, C., Peng, L. & Yu, G. Conductive “Smart” hybrid hydrogels with PNIPAM and nanostructured conductive polymers. Adv. Funct. Mater. 25 , 1219–1225 (2015).
Zhao, Y. et al. Somatosensory actuator based on stretchable conductive photothermally responsive hydrogel. Sci. Robot. 6 , 1–12 (2021).
Rich, S. I., Wood, R. J. & Majidi, C. Untethered soft robotics. Nat. Electron. 1 , 102–112 (2018).
Tawfick, S. & Tang, Y. Stronger artificial muscles, with a twist. Science 365 , 125–126 (2019).
Miriyev, A., Stack, K. & Lipson, H. Soft material for soft actuators. Nat. Commun. 8 , 1–8 (2017).
Huber, J. E., Fleck, N. A. & Ashby, M. F. The selection of mechanical actuators based on performance indices. Proc. R. Soc. Lond. Ser. A Math. Phys. Eng. Sci. 453 , 2185–2205 (1997).
Saghaian, S. M., Karaca, H. E., Souri, M., Turabi, A. S. & Noebe, R. D. Tensile shape memory behavior of Ni50.3Ti29.7Hf20 high temperature shape memory alloys. Mater. Des. 101 , 340–345 (2016).
Kornbluh, R. et al. Application of Dielectric Elastomer EAP Actuators. in Electroactive Polymer (EAP) Actuators as Artificial Muscles: Reality, Potential, and Challenges, Second Edition (ed Bar-Cohen, Y.) 529–581 (SPIE, 2004). https://doi.org/10.1117/3.547465.ch16 .
Guo, J., Wang, Z., Tong, L., Lv, H. & Liang, W. Shape memory and thermo-mechanical properties of shape memory polymer/carbon fiber composites. Compos. Part A Appl. Sci. Manuf. 76 , 162–171 (2015).
Yang, D. et al. Buckling pneumatic linear actuators inspired by muscle. Adv. Mater. Technol. 1 , 1600055 (2016).
Racles, C., Cazacu, M., Fischer, B. & Opris, D. M. Synthesis and characterization of silicones containing cyanopropyl groups and their use in dielectric elastomer actuators. Smart Mater. Struct. 22 , 104004 (2013).
Peng, Z., Shi, Y., Chen, N., Li, Y. & Pei, Q. Stable and high-strain dielectric elastomer actuators based on a carbon nanotube-polymer bilayer electrode. Adv. Funct. Mater. 31 , 2008321 (2021).
La, T.-G., Lau, G.-K., Shiau, L.-L. & Wei-Yee Tan, A. Muscle-like high-stress dielectric elastomer actuators with oil capsules. Smart Mater. Struct. 23 , 105006 (2014).
Ji, Z., Yan, C., Yu, B., Wang, X. & Zhou, F. Multimaterials 3D printing for free assembly manufacturing of magnetic driving soft actuator. Adv. Mater. Interfaces 4 , 1–6 (2017).
Zhang, J. et al. Liquid crystal elastomer‐based magnetic composite films for reconfigurable shape‐morphing soft miniature machines. Adv. Mater. 33 , 2006191 (2021).
Lee, J. G. & Rodrigue, H. Efficiency of origami-based vacuum pneumatic artificial muscle for off-grid operation. Int. J. Precis. Eng. Manuf. Green. Technol. 6 , 789–797 (2019).
Do, T. N., Phan, H., Nguyen, T. & Visell, Y. Miniature soft electromagnetic actuators for robotic applications. Adv. Funct. Mater. 28 , 1800244 (2018).
Shang, J. et al. A single-port robotic system for transanal microsurgery-design and validation. IEEE Robot. Autom. Lett. 2 , 1510–1517 (2017).
Zhang, D., Chen, J., Li, W., Bautista Salinas, D. & Yang, G. Z. A microsurgical robot research platform for robot-assisted microsurgery research and training. Int. J. Comput. Assist. Radiol. Surg. 15 , 15–25 (2020).
Xi, W. et al. Rolled-up magnetic microdrillers: towards remotely controlled minimally invasive surgery. Nanoscale 5 , 1294–1297 (2013).
Barbot, A., Power, M., Seichepine, F. & Yang, G.-Z. Liquid seal for compact micropiston actuation at the capillary tip. Sci. Adv. 6 , eaba5660 (2020).
Chee, P. S., Minjal, M. N., Leow, P. L. & Ali, M. S. M. Wireless powered thermo-pneumatic micropump using frequency-controlled heater. Sens. Actuators A Phys. 233 , 1–8 (2015).
Nam, D. N. C. & Ahn, K. K. Design of an IPMC diaphragm for micropump application. Sens. Actuators A Phys. 187 , 174–182 (2012).
McDaid, A. J., Aw, K. C., Haemmerle, E. & Xie, S. Q. Control of IPMC actuators for microfluidics with adaptive “Online” iterative feedback tuning. IEEE/ASME Trans. Mechatron. 17 , 789–797 (2012).
Marette, A. et al. Flexible zinc-tin oxide thin film transistors operating at 1 kV for integrated switching of dielectric elastomer actuators arrays. Adv. Mater. 29 , 1700880 (2017).
Diller, E. & Sitti, M. Micro-scale mobile robotics. Found. Trends Robot. 2 , 143–259 (2011).
Wautelet, M. Scaling laws in the macro-, micro- and nanoworlds. Eur. J. Phys. 22 , 601–611 (2001).
Yan, G., Ye, D., Zan, P., Wang, K. & Ma, G. Micro-robot for endoscope based on wireless power transfer. in Proc. 2007 IEEE International Conference on Mechatronics and Automation. 3577–3581 (2007).
Aldhaher, S., Mitcheson, P. D., Arteaga, J. M., Kkelis, G. & Yates, D. C. Light-weight wireless power transfer for mid-air charging of drones. 2017 11th Eur. Conf. Antennas Propagation, EUCAP 2017336–2017340 (2017) https://doi.org/10.23919/EuCAP.2017.7928799 .
Bandari, V. K. et al. A flexible microsystem capable of controlled motion and actuation by wireless power transfer. Nat. Electron. 3 , 172–180 (2020).
Mitcheson, P. D., Yeatman, E. M., Rao, G. K., Holmes, A. S. & Green, T. C. Energy harvesting from human and machine motion for wireless electronic devices. Proc. IEEE 96 , 1457–1486 (2008).
Ryu, H., Yoon, H. J. & Kim, S. W. Hybrid energy harvesters: toward sustainable energy harvesting. Adv. Mater. 31 , 1802898 (2019).
Lee, T. D. & Ebong, A. U. A review of thin film solar cell technologies and challenges. Renew. Sustain. Energy Rev. 70 , 1286–1297 (2017).
Green, M. A. et al. Solar cell efficiency tables (version 54). Prog. Photovolt. Res. Appl. 27 , 565–575 (2019).
Wei, C. & Jing, X. A comprehensive review on vibration energy harvesting: modelling and realization. Renew. Sustain. Energy Rev. 74 , 1–18 (2017).
Article MathSciNet Google Scholar
Zhu, J. et al. Stretchable wideband dipole antennas and rectennas for RF energy harvesting. Mater. Today Phys. 18 , 100377 (2021).
Yin, L. et al. A passive perspiration biofuel cell: high energy return on investment. Joule 5 , 1888–1904 (2021).
Zhang, X. S. et al. All-in-one self-powered flexible microsystems based on triboelectric nanogenerators. Nano Energy 47 , 410–426 (2018).
Ding, W., Wang, A. C., Wu, C., Guo, H. & Wang, Z. L. Human-machine interfacing enabled by triboelectric nanogenerators and tribotronics. Adv. Mater. Technol. 4 , 1800487 (2019).
Download references
Acknowledgements
The authors would like to acknowledge the financial support of the EPSRC program Grant (EP/P012779/1).
Author information
Authors and affiliations.
Department of Electrical and Electronic Engineering, Imperial College London, Exhibition Road, London, SW7 2AZ, UK
Mayue Shi & Eric M. Yeatman
You can also search for this author in PubMed Google Scholar
Contributions
E.M.Y. lead and supervised this review and conceived the concept of energy and actuation management. M.S. consolidated the data from the literature and prepared the original draft, figures, and tables. E.M.Y. and M.S. revised the paper. Both authors have read and agreed to the published version of the paper.
Corresponding author
Correspondence to Mayue Shi .
Ethics declarations
Conflict of interest.
The authors declare that they have no conflicts of interest.
Rights and permissions
Open Access This article is licensed under a Creative Commons Attribution 4.0 International License, which permits use, sharing, adaptation, distribution and reproduction in any medium or format, as long as you give appropriate credit to the original author(s) and the source, provide a link to the Creative Commons license, and indicate if changes were made. The images or other third party material in this article are included in the article’s Creative Commons license, unless indicated otherwise in a credit line to the material. If material is not included in the article’s Creative Commons license and your intended use is not permitted by statutory regulation or exceeds the permitted use, you will need to obtain permission directly from the copyright holder. To view a copy of this license, visit http://creativecommons.org/licenses/by/4.0/ .
Reprints and permissions
About this article
Cite this article.
Shi, M., Yeatman, E.M. A comparative review of artificial muscles for microsystem applications. Microsyst Nanoeng 7 , 95 (2021). https://doi.org/10.1038/s41378-021-00323-5
Download citation
Received : 02 August 2021
Revised : 26 September 2021
Accepted : 05 October 2021
Published : 23 November 2021
DOI : https://doi.org/10.1038/s41378-021-00323-5
Share this article
Anyone you share the following link with will be able to read this content:
Sorry, a shareable link is not currently available for this article.
Provided by the Springer Nature SharedIt content-sharing initiative
This article is cited by
A strong and fast millimeter-sized soft pneumatic actuator based on alternative pole water electrolysis.
- Hadi Kolivand
- Azita Souri
- Arash Ahmadi
International Journal of Intelligent Robotics and Applications (2024)
Advanced ionic actuators with high-performance and high-reproducibility based on free-standing bacterial cellulose-reinforced poly(diallyldimethylammonium chloride) membranes and PEDOT/PSS electrodes
- Qinchuan Li
Cellulose (2023)
Quick links
- Explore articles by subject
- Guide to authors
- Editorial policies

On the Role of “Muscle Memory” in Interaction Design
- Conference paper
- First Online: 08 July 2020
- Cite this conference paper
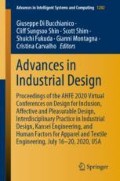
- Miao Liu 20 &
- Xingchun Yang 20
Part of the book series: Advances in Intelligent Systems and Computing ((AISC,volume 1202))
Included in the following conference series:
- International Conference on Applied Human Factors and Ergonomics
2947 Accesses
1 Citations
“Muscle memory” refers to the human body’s muscles have memory effects. After the same action is repeated many times, the muscles will form conditioned reflexes. With the development of screen technology, after more and more physical buttons are replaced with screen operations, the structure of the machine no longer follows its functions to design, especially some button designs. This poses new challenges to the accuracy, timeliness, and reduction of mis-operation rates. This article studies different interface designs and proposes several interface design principles that are consistent with muscle memory. Based on this principle, an APP launcher based on “muscle memory” is produced to verify whether the criterion is reasonable. This article links “muscle memory” with interoperability, and aims to create, build, and facilitate convenient relationships between people and products and services. A feasible direction is proposed for exploring better human interaction experience.
This is a preview of subscription content, log in via an institution to check access.
Access this chapter
- Available as PDF
- Read on any device
- Instant download
- Own it forever
- Available as EPUB and PDF
- Compact, lightweight edition
- Dispatched in 3 to 5 business days
- Free shipping worldwide - see info
Tax calculation will be finalised at checkout
Purchases are for personal use only
Institutional subscriptions
Krakauer, J.W., Shadmehr, R.: Consolidation of motor memory. Trends Neurosci. 29 (1), 58–64 (2006)
Article Google Scholar
Ma, L., et al.: Changes in regional activity are accompanied with changes in inter-regional connectivity during 4 weeks motor learning. Brain Res. 1318 , 64–76 (2010)
Lee, D.T., Schmidt, A.R.: Motor Control and Learning: A Behavioural Emphasis, 4th edn. Human Kinetics, Windsor (2005)
Google Scholar
Download references
Acknowledgments
We thank everyone who provided valuable suggestions and feedback during the writing of this paper. Thanks to the supported by Shanghai Summit Discipline in Design - DA17014.
Author information
Authors and affiliations.
East China University of Science and Technology, Shanghai, 200237, People’s Republic of China
Miao Liu & Xingchun Yang
You can also search for this author in PubMed Google Scholar
Corresponding author
Correspondence to Xingchun Yang .
Editor information
Editors and affiliations.
Dipartimento di Architettura, Universitàdegli Studi G.d’Annunzio”, Pescara, Pescara, Italy
Giuseppe Di Bucchianico
Department of Industrial Design, University of Illinois at Urbana Champaign, Champaign, IL, USA
Cliff Sungsoo Shin
University of Notre Dame, Indiana, IN, USA
System Design and Management, Keio University, Tokyo, Japan
Shuichi Fukuda
Faculty of Architecture, University of Lisbon, Lisboa, Portugal
Gianni Montagna
Cristina Carvalho
Rights and permissions
Reprints and permissions
Copyright information
© 2020 The Editor(s) (if applicable) and The Author(s), under exclusive license to Springer Nature Switzerland AG
About this paper
Cite this paper.
Liu, M., Yang, X. (2020). On the Role of “Muscle Memory” in Interaction Design. In: Di Bucchianico, G., Shin, C., Shim, S., Fukuda, S., Montagna, G., Carvalho, C. (eds) Advances in Industrial Design. AHFE 2020. Advances in Intelligent Systems and Computing, vol 1202. Springer, Cham. https://doi.org/10.1007/978-3-030-51194-4_83
Download citation
DOI : https://doi.org/10.1007/978-3-030-51194-4_83
Published : 08 July 2020
Publisher Name : Springer, Cham
Print ISBN : 978-3-030-51193-7
Online ISBN : 978-3-030-51194-4
eBook Packages : Engineering Engineering (R0)
Share this paper
Anyone you share the following link with will be able to read this content:
Sorry, a shareable link is not currently available for this article.
Provided by the Springer Nature SharedIt content-sharing initiative
- Publish with us
Policies and ethics
- Find a journal
- Track your research
Help | Advanced Search
Computer Science > Computation and Language
Title: leave no context behind: efficient infinite context transformers with infini-attention.
Abstract: This work introduces an efficient method to scale Transformer-based Large Language Models (LLMs) to infinitely long inputs with bounded memory and computation. A key component in our proposed approach is a new attention technique dubbed Infini-attention. The Infini-attention incorporates a compressive memory into the vanilla attention mechanism and builds in both masked local attention and long-term linear attention mechanisms in a single Transformer block. We demonstrate the effectiveness of our approach on long-context language modeling benchmarks, 1M sequence length passkey context block retrieval and 500K length book summarization tasks with 1B and 8B LLMs. Our approach introduces minimal bounded memory parameters and enables fast streaming inference for LLMs.
Submission history
Access paper:.
- HTML (experimental)
- Other Formats
References & Citations
- Google Scholar
- Semantic Scholar
BibTeX formatted citation

Bibliographic and Citation Tools
Code, data and media associated with this article, recommenders and search tools.
- Institution
arXivLabs: experimental projects with community collaborators
arXivLabs is a framework that allows collaborators to develop and share new arXiv features directly on our website.
Both individuals and organizations that work with arXivLabs have embraced and accepted our values of openness, community, excellence, and user data privacy. arXiv is committed to these values and only works with partners that adhere to them.
Have an idea for a project that will add value for arXiv's community? Learn more about arXivLabs .

An official website of the United States government
The .gov means it’s official. Federal government websites often end in .gov or .mil. Before sharing sensitive information, make sure you’re on a federal government site.
The site is secure. The https:// ensures that you are connecting to the official website and that any information you provide is encrypted and transmitted securely.
- Publications
- Account settings
Preview improvements coming to the PMC website in October 2024. Learn More or Try it out now .
- Advanced Search
- Journal List
- Front Psychol
Memory: An Extended Definition
Gregorio zlotnik.
1 Clinique de la Migraine de Montreal, Montreal, QC, Canada
Aaron Vansintjan
2 Department of Film, Media and Cultural Studies, Birkbeck, University of London, London, United Kingdom
Recent developments in science and technology point to the need to unify, and extend, the definition of memory. On the one hand, molecular neurobiology has shown that memory is largely a neuro-chemical process, which includes conditioning and any form of stored experience. On the other hand, information technology has led many to claim that cognition is also extended, that is, memory may be stored outside of the brain. In this paper, we review these advances and describe an extended definition of memory. This definition is largely accepted in neuroscience but not explicitly stated. In the extended definition, memory is the capacity to store and retrieve information. Does this new definition of memory mean that everything is now a form of memory? We stress that memory still requires incorporation, that is, in corpore . It is a relationship – where one biological or chemical process is incorporated into another, and changes both in a permanent way. Looking at natural and biological processes of incorporation can help us think of how incorporation of internal and external memory occurs in cognition. We further argue that, if we accept that there is such a thing as the storage of information outside the brain – and that this organic, dynamic process can also be called “memory” – then we open the door to a very different world. The mind is not static. The brain, and the memory it uses, is a work in progress; we are not now who we were then.
Introduction
In the short story “Funes, the memorious,” Jorge Luis Borges invites us to imagine a man, Funes, who cannot forget anything. The narrator is ashamed in the inexactness of his retelling: his own memory is “remote and weak,” in comparison to that of his subject, which resembles “a stammering greatness.” Unlike Funes, he says, “we all live by leaving behind” – life is impossible without forgetting. He goes on to note that, even though Funes could remember every split second, he couldn’t classify or abstract from his memories. “To think is to forget a difference, to generalize, to abstract.” The reader may be led to wonder how Funes’ brain has the capacity to store all of that memory. doesn’t it reach its limits at some point? Borges leaves that question to our imagination.
In popular culture, memory is often thought of as some kind of physical thing that is stored in the brain; a subjective, personal experience that we can recall at will. This way of thinking about memory has led many to wonder if there is a maximum amount of memories we can have. But, this idea of memory is at odds with advances in the science of memory over the last century: memory isn’t really a fixed thing stored in the brain, but is more of a chemical process between neurons, which is not static. What’s more, advances in information technology are pushing our understanding of memory into new directions. We now talk about memory on a hard drive, or as a chemical change between neurons. Yet, these different definitions of memory continue to co-exist. A more narrow definition of memory, as the storage of experiences in the brain, is increasingly at odds with an extended definition, which acknowledges these advances. However, while this expanded definition is often implicitly used, it is rarely explicitly acknowledged or stated. Today, the question is no longer, how many memories can we possibly have, but, how is the vast amount of memory we process on a daily basis integrated into cognition?
In this paper, we outline these advances and the currently accepted definitions of memory, arguing that these necessarily imply that we should today adopt an extended definition. In the following, we first describe some key advances in the science of memory, cognitive theory, and information technology. These suggest to us that we are already using a unified, and extended, definition of memory, but rarely made explicit. Does this new definition of memory mean that everything is now a form of memory? We argue that looking at natural and biological processes of incorporation can help us think of how incorporation of internal and external memory occurs in cognition. Finally, we note some of the implications of this extended definition of memory.
Background: Advances in the Science of Memory
Already in the 19th century, the recognition that the number of neurons in the brain doesn’t increase significantly after reaching adulthood suggested to early neuroanatomists that memories aren’t primarily stored through the creation of neurons, but rather through the strengthening of connections between neurons ( Ramón y Cajal, 1894 ). In 1966, the breakthrough discovery of long-term potentiation (LTP) suggested that memories may be encoded in the strength of synaptic signals between neurons ( Bliss and Lømo, 1973 ). And so we started understanding memory as a neuro-chemical process. The studies by Eric Kandel of the Aplysia californica , for which he won the Nobel prize, for example, show that classical conditioning is a basic form of memory storage and is observable on a molecular level within simple organisms ( Kandel et al., 2012 ). This in effect expanded the definition of memory to include storage of information in the neural networks of simple lifeforms. Increasingly, researchers are exploring the chemistry behind memory development and recall, suggesting these molecular processes can lead to psychological adaptations (e.g., Coderre et al., 2003 ; Laferrière et al., 2011 ).
Memory is today defined in psychology as the faculty of encoding, storing, and retrieving information ( Squire, 2009 ). Psychologists have found that memory includes three important categories: sensory, short-term, and long-term. Each of these kinds of memory have different attributes, for example, sensory memory is not consciously controlled, short-term memory can only hold limited information, and long-term memory can store an indefinite amount of information.
Key to the emerging science of memory is the question of how memory is consolidated and processed. Long-term storage of memories happens on a synaptic level in most organisms ( Bramham and Messaoudi, 2005 ), but, in complex organisms like ourselves, there is also a second form of memory consolidation: systems consolidation moves, processes, and more permanently stores memories ( Frankland and Bontempi, 2005 ). Today, there are many models of how memory is consolidated in cognition. Single-system models posit that the hippocampus supports the neocortex in encoding and storing long-term memories through strengthening connections, finally leading the memory to become independent from the hippocampus (Ibid.). Multiple-trace theory instead proposes that each memory has a unique code or memory trace, which continues to involve the hippocampus to an extent ( Hintzman and Block, 1971 ; Hintzman, 1986 , 1990 ; Whittlesea, 1987 ; Versace et al., 2014 ; Briglia et al., 2018 ). In another theory, memory is understood as a form of negative entropy or rich energy ( Wiener, 1961 , 1988 ), which is then processed in a way that minimizes the expenditure of energy by the brain ( Friston, 2010 ; Van der Helm, 2016 ). Our heightened capacity to store information may be due to our ability to reduce disorder and process large amounts of information rapidly, a necessarily non-linear process ( Wiener, 1961 , 1988 ). The forgetting and fading of memories is also understood as being an important aspect of the functioning and utility of these memories ( Staniloiu and Markowitsch, 2012 ). As with a computer hard drive, memories can also be “corrupted” – false memories are commonly studied within forensic psychology ( Loftus, 2005 ). Together, these advances highlight how different kinds of memory storage are non-linear – that is, subject to complex systems interactions – contextual, and plastic. They also shed light on why, and how, we are able to live with such large quantities of information. It may not be that Funes has the special ability to remember everything, but that he lacks our ability to incorporate, and sort through, a potentially infinite amount of information.
The advance of the fields of genetics and epigenetics has also given us new metaphors to describe memory. We understand DNA as a structure that carries information that we call “genetic code” – kind of like a computer chip for biological processes. Today, the metaphor has come full circle and we can now use DNA to store and extract digital data ( Church et al., 2012 ). The study of epigenetics suggests that simple lifeforms pass on memories across generations through genetic code ( Klosin et al., 2017 ; Posner et al., 2019 ), suggesting a need to study whether humans and other complex life forms may do so as well. With these advances, our understanding of how memory is stored has expanded once again.
Further, we can now store memory in places that we haven’t been able to before. Smartphones, mind-controlled prosthetic limbs, and Google Glasses all offer new ways to store information and thereby interact with our surroundings. Our ability to produce information alters how we perceive the world, with far-reaching implications. As Stephen Hawking, the Nobel prize-winning physicist explained in his 1996 lecture, “Life in the universe,”
What distinguishes us from [our ancestors], is the knowledge that we have accumulated over the last 10000 years, and particularly, over the last three hundred. I think it is legitimate to take a broader view, and include externally transmitted information, as well as DNA, in the evolution of the human race ( Hawking, 1996 ).
The sheer quantity of available information today, as well as developments in an understanding of memory – from fixed and physical to dynamic, chemical, and a process of rich energy transfer – lead to a very different picture of memory than the one we had 100 years ago. Memory seems to exist everywhere, from an Aplysia ’s ganglion to DNA to a hard drive.
To account for these developments, cognitive scientists now propose that human cognition is actually extended beyond the brain in ways that theories of the mind did not previously recognize ( Clark and Chalmers, 1998 ; Clark, 2008 ). This approach is being called 4E cognition (Embodied, Embedded, Extended, and Enactive). For example, enactivism posits that cognition is a dynamic interaction between an organism and its environment ( Varela et al., 1991 ; Chemero, 2009 ; Menary, 2010 ; Rowlands, 2010 ; Favela and Chemero, 2016 ; Briglia et al., 2018 ). According to this framework, cognition is a process of incorporation between the environment and the body/brain/mind. To be clear, cognition is not incorporated in the surroundings, only the corpus can incorporate, and thus cognition (or what we call “mind”) is a product of the interaction between the brain, the body, and the environment.
Extending Memory
These developments indicate that we need to reconceptualize our definition of memory. What is the difference between trying to recall a childhood experience, and searching for an important email archived years ago? This distinction is best represented through the difference in how we use the words “memory” and “memories.” Usually, “memories” tends to refer to events recalled from the past, which are seen as more representational and subjective. In contrast, “memory” now is used to refer to storage of information in general , including in DNA, digital information storage, and neuro-chemical processes. Today, science has moved far beyond a popular understanding of memory as fixed, subjective, and personal. In the extended definition, it is simply the capacity to store and retrieve information . To illustrate why memory has extended beyond this original use, we want to ask the reader: what do a stressed-out driver and a snail have in common?
(1). A homeowner has been trying to sell her house for a year, and worrying about it. One day, she’s driving to work and becomes extremely anxious, for no apparent reason. She wasn’t thinking of anything in particular at the time. Confused, she looks around, and notices a billboard advertising a real estate agency. She realizes that she had seen it out of the corner of her eye, and her brain had then processed the information while she was thinking of something else, which then triggered the anxiety attack.
(2). Consider a nerve cell of an A. californica , a kind of sea snail, which is prodded vigorously for a short time period, provoking an immediate withdrawal response. Shortly afterward, it is prodded less intensely, but, it elicits the same withdrawal response. It is found that the slugs’ nerve cell is sensitive for up to 24 h – the nerve cells “remember” past pain.
Each example illustrates a different kind of chemical, biological process. In the first example, an outside stimulus triggers a stress response for the homeowner. We can surmise that though she didn’t “remember” anything, non-consciously, she did. In the second example, the snail certainly “remembers” the provocation, even though this memory is only stored in a few cells. But can we really call this memory?
However, on closer examination, we are forced to concede that each of them should be called a form of memory. First, consider the homeowner: her brain “remembers” something that does not occur to her as a conscious thought. It is clearly a chemical process occurring in the background. Most would grant that this would nevertheless be a form of memory, as it involves recalling information stored in her brain. Already, a broader definition of memory is used that does not imply conscious attention. Now, consider the snail: it is also storing information chemically. Once again, this does not involve a conscious, subjective process of storing and remembering – it is purely reactive, but information is being stored and recalled nonetheless. We would need to concede that if the homeowner’s experience counts as memory, then the slug’s automatic response does as well. There is in fact little difference between the first two examples: there is a transfer of information that causes a reaction. Both should be considered forms of memory.
A Slippery Slope?
If we agree with this expanded definition of memory, then it follows that experience is also a form of stored information, kinds of memory . We are not saying that a particular experience, as an event , is a memory. Rather, we here use the word “experience” as connoted by the phrase “an experienced driver,” an “experienced writer.” They have a set of experiences, remembered through practice, and retrieved when they drive, or write. When we accumulate knowledge, information, and techniques, then the accumulation of those separate processes constitute experience . This experience involves retrieval of information, conversely, being experienced is the process of retrieving memory.
Under this definition, even immunological and allergy processes may be considered memory. There is a storage of information of the allergen or the viral/bacterial aggressor and when the aggressor or allergen re-appears there is a cascade of inflammatory processes. This can be considered the storage and retrieval of information, and thus a form of memory. This does not contradict the accepted definition of memory within psychology, as it is still seen as the ability to encode, store, and recall information. Rather, it extends it to processes not just bound by the brain.
If memory is indeed defined as “the capacity to store and/or retrieve information,” then this may lead anyone to ask – what isn’t memory? Wouldn’t this definition of memory be far too broad, and include a vast range of phenomena? Is the extended definition of memory, as is being proposed by neurobiologists and cognitive theorists, a slippery slope?
As we suggested above, however, memory still involves a process of incorporation, that is, requiring a corpus . While memory may be stored on the cloud, it requires a system of incorporation with the body and therefore the mind. In other words, the “cloud” by itself is not memory, but operates through an infrastructure (laptops, smart phones, Google Glasses) that are integrated with the brain-mind through learned processes of storage and recall. The conditioning of an Aplysia ’s ganglion is incorporated into an organism. Memory, it seems, is not just mechanistic, but a dynamic process. It is a relationship – where one biological or chemical process is incorporated into another, and changes both in a permanent way. A broadened definition must account for this dynamic relationship between organisms and their environment.
How can we understand this process of incorporation? It appears that symbiotic incorporation of biological processes is quite common in nature. Recent studies offer more evidence that early cells acquired mitochondria by, at some point, incorporating external organisms into their own cell structure ( Thrash et al., 2011 ; Ferla et al., 2013 ). Mitochondria have their own genome, which is similar to that of bacteria. What was once a competitor and possibly a parasite became absorbed into the organism – and yet, the mitochondrion was not fully incorporated and retains many of its own processes of self-organization and memory storage, separate from the cell it resides in. This evolutionary process highlights the way by which external properties may become incorporated into the internal, changing both. Looking at natural and biological processes of incorporation can help us think of how incorporation of internal and external memory occurs in cognition.
Implications
This extended definition of memory may seem ludicrous and hard to accept. You may be tempted to throw up your hands and go back to the old, restricted, definition of memory – one that requires the transmission of subjective memories.
We beg you not to. There are several benefits of this approach to memory. First, in biology, expanding the definition of memory helps us shift from a focus on “experience” (which suggests an immaterial event) to a more material phenomenon: a deposit of events that may be stored and used afterward. By expanding the concept of memory, the study of memory within molecular neurobiology becomes more relevant and important. This expanded definition is in large part already widely accepted, for example, in Kandel’s Aplysia , conditioning is acknowledged to be a part of memory, and memory is not a part of conditioning. Memory would become the umbrella for learning, conditioning, and other processes of the mind/brain. Doing so changes the frame of observation from one which understands memory as a narrow, particular process, to one which understands it as a dynamic, fluid, and interactive phenomenon, neither just chemical or digital but integrated into our experience through multiple media. Second, it helps to conceptualize the relationship between biology, psychology, cognitive science, and computer science – as all three involve studying the transfer of information.
Third, it opens up an interesting way to imagine our own future. If we accept that there is such a thing as the storage of information outside the brain – and that this organic, dynamic process can also be called “memory” – then we open the door to a very different world. The mind is not static. Rather, like early cells acquiring mitochondria, it incorporates information from its surroundings, which in turn changes it. The brain, and the memory it uses, is a work in progress; we are not now who we were then. Many have already noted the extent to which we are cyborgs ( Harraway, 1991 ; Clark, 2003 , 2005 ); this neat line between human and technology may become more and more blurred as we develop specialized tools to store all kinds of information in our built environment. In what ways will the mind-brain function differently as it becomes increasingly more incorporated in its milieu, relying on it for information storage and processing?
Now let’s talk about Funes. His inability to forget his memories may seem familiar to some, a metaphor for our current condition. We may now recognize a bit of ourselves in him: we don’t see limits in our capacity to store new information, and the sheer availability of it is sometimes overwhelming. Even without the arrival of the Information Age, we carry with us through life a heavy load of disappointments, broken dreams, little tragedies and many memories. We know that forgetting is a must and a challenge. Yet, we are learning rapidly how to incorporate and use the massive amounts of data now available to us. The main challenge for each of us is to harness and control the unleashed powers given to us by technology. The future is uncertain, but some things remain the same. As Kandel (2007 , p. 10) wrote, “We are who we are in great measure because of what we learn, and what we remember.”
Author Contributions
GZ and AV drafted and edited the manuscript. Both authors contributed to manuscript revision, read, and approved the submitted version.
Conflict of Interest
The authors declare that the research was conducted in the absence of any commercial or financial relationships that could be construed as a potential conflict of interest.
Acknowledgments
The authors wish to thank Michael Lifshitz, Ph.D. for reading an early copy of this article and providing feedback. The authors also wish to thank Steven J. Lynn, Alan M. Rapoport, and Morgan Craig for the feedback and encouragement.
- Bliss T., Lømo T. (1973). Long-lasting potentiation of synaptic transmission in the dentate area of the anaesthetized rabbit following stimulation of the perforant path. J. Physiol. 232 331–356. 10.1113/jphysiol.1973.sp010273 [ PMC free article ] [ PubMed ] [ CrossRef ] [ Google Scholar ]
- Bramham C. R., Messaoudi E. (2005). BDNF function in adult synaptic plasticity: the synaptic consolidation hypothesis. Prog. Neurobiol. 76 99–125. 10.1016/j.pneurobio.2005.06.003 [ PubMed ] [ CrossRef ] [ Google Scholar ]
- Briglia J., Servajean P., Michalland A. H., Brunel L., Brouillet D. (2018). Modeling an enactivist multiple-trace memory. ATHENA: a fractal model of human memory. J. Math. Psychol. 82 97–110. 10.1016/j.jmp.2017.12.002 [ CrossRef ] [ Google Scholar ]
- Chemero A. (2009). Radical Embodied Cognitive Science. Cambridge, MA: MIT Press. [ Google Scholar ]
- Church G. M., Gao Y., Kosuri S. (2012). Next-generation digital information storage in DNA. Science 337 : 1628 . 10.1126/science.1226355 [ PubMed ] [ CrossRef ] [ Google Scholar ]
- Clark A. (2003). Natural-Born Cyborgs: Minds, Technologies, and the Future of Human Intelligence. New York, NY: Oxford University Press. [ Google Scholar ]
- Clark A. (2005). “Intrinsic content, active memory and the extended mind”. Analysis 65 1–11. 10.1111/j.1467-8284.2005.00514.x [ CrossRef ] [ Google Scholar ]
- Clark A. (2008). Supersizing the Mind: Embodiment, Action, and Cognitive Extension OUP. Oxford: Oxford University Press. [ Google Scholar ]
- Clark A., Chalmers D. (1998). The extended mind. Analysis 58 7–19. [ Google Scholar ]
- Coderre T. J., Mogil J. S., Bushnell M. C. (2003). “ The biological psychology of pain ,” in Handbook of Psychology , eds Gallagher M., Nelson R. J. (New York, NY: Wiley; ), 237–268. [ Google Scholar ]
- Favela L. H., Chemero A. (2016). “ The animal-environment system ,” in Foundations of Embodied Cognition: Perceptual and Emotional Embodiment , eds Coello Y., Fischer M. H. (New York, NY: Routledge/Taylor & Francis Group; ), 59–74. [ Google Scholar ]
- Ferla M. P., Thrash J. C., Giovannoni S. J., Patrick W. M. (2013). New rRNA gene-based phylogenies of the Alphaproteobacteria provide perspective on major groups, mitochondrial ancestry and phylogenetic instability. PLoS One 8 : e83383 . 10.1371/journal.pone.0083383 [ PMC free article ] [ PubMed ] [ CrossRef ] [ Google Scholar ]
- Frankland P. W., Bontempi B. (2005). The organization of recent and remote memories. Nat. Rev. Neurosci. 6 119–130. 10.1038/nrn1607 [ PubMed ] [ CrossRef ] [ Google Scholar ]
- Friston K. (2010). The free-energy principle: a unified brain theory? Nat. Rev. Neurosci. 11 127–138. 10.1038/nrn2787 [ PubMed ] [ CrossRef ] [ Google Scholar ]
- Harraway D. J. (1991). Simians, Cyborgs, and Women: The Reinvention of Nature . New York: Routledge. [ Google Scholar ]
- Hawking S. (1996). Life in the Universe. Available at: http://www.hawking.org.uk/life-in-the-universe.html (accessed February 2, 2019). [ Google Scholar ]
- Hintzman D. L. (1986). “Schema abstraction” in a multiple-trace memory model. Psychol. Rev. 93 411–428. 10.1037//0033-295x.93.4.411 [ CrossRef ] [ Google Scholar ]
- Hintzman D. L. (1990). Human learning and memory: connections and dissociations. Annu. Rev. Psychol. 41 109–319. [ PubMed ] [ Google Scholar ]
- Hintzman D. L., Block R. A. (1971). Repetition and memory: evidence for a multiple trace hypothesis. J. Exp. Psychol. 88 297–306. 10.1037/h0030907 [ CrossRef ] [ Google Scholar ]
- Kandel E. R. (2007). In Search of Memory: The Emergence of a New Science of Mind. New York, NY: WW Norton & Company. [ Google Scholar ]
- Kandel E. R., Schwartz J. H., Jessell T. M., Siegelbaum S. A., Hudspeth A. J. (2012). Principles of Neural Science , 5th Edn New York, NY: McGraw-Hill. [ Google Scholar ]
- Klosin A., Casas E., Hidalgo-Carcedo C., Vavouri T., Lehner B. (2017). Transgenerational transmission of environmental information in C. elegans. Science 356 320–323. 10.1126/science.aah6412 [ PubMed ] [ CrossRef ] [ Google Scholar ]
- Laferrière A., Pitcher M. H., Haldane A., Huang Y., Cornea V., Kumar N., et al. (2011). PKMζ is essential for spinal plasticity underlying the maintenance of persistent pain. Mol. Pain 7 : 99 . 10.1186/1744-8069-7-99 [ PMC free article ] [ PubMed ] [ CrossRef ] [ Google Scholar ]
- Loftus E. F. (2005). Planting misinformation in the human mind: a 30-year investigation of the malleability of memory. Learn. Mem. 12 361–366. 10.1101/lm.94705 [ PubMed ] [ CrossRef ] [ Google Scholar ]
- Menary R. (2010). Introduction to the special issue on 4E cognition. Phenomenol. Cogn. Sci. 9 459–463. 10.1007/s11097-010-9187-6 [ CrossRef ] [ Google Scholar ]
- Posner R., Toker I. A., Antonova O., Star E., Anava S., Azmon E., et al. (2019). Neuronal small RNAs control behavior transgenerationally. Cell 177 : 1814-1826.e15 . 10.1016/j.cell.2019.04.029 [ PMC free article ] [ PubMed ] [ CrossRef ] [ Google Scholar ]
- Ramón y Cajal S. (1894). The croonian lecture: la fine structure des centres nerveux. Proc. R. Soc. Lond. 55 444–468. 10.1098/rspl.1894.0063 [ CrossRef ] [ Google Scholar ]
- Rowlands M. (2010). The New Science of the Mind: From Extended Mind to Embodied Phenomenology. Cambridge, MA: MIT Press. [ Google Scholar ]
- Squire L. R. (2009). Memory and brain systems: 1969–2009. J. Neurosci. 29 12711–12716. 10.1523/jneurosci.3575-09.2009 [ PMC free article ] [ PubMed ] [ CrossRef ] [ Google Scholar ]
- Staniloiu A., Markowitsch H. J. (2012). Towards solving the riddle of forgetting in functional amnesia: recent advances and current opinions. Front. Psychol. 3 : 403 . 10.3389/fpsyg.2012.00403 [ PMC free article ] [ PubMed ] [ CrossRef ] [ Google Scholar ]
- Thrash J. C., Boyd A., Huggett M. J., Grote J., Carini P., Yoder R. J., et al. (2011). Phylogenomic evidence for a common ancestor of mitochondria and the SAR11 clade. Sci. Rep. 1 : 13 . 10.1038/srep00013 [ PMC free article ] [ PubMed ] [ CrossRef ] [ Google Scholar ]
- Van der Helm P. A. (2016). Structural coding versus free-energy predictive coding. Psychonom. Bull. Rev. 23 663–677. 10.3758/s13423-015-0938-9 [ PubMed ] [ CrossRef ] [ Google Scholar ]
- Varela F. J., Thompson E., Rosch E. (1991). The Embodied Mind: Cognitive Science and Human Experience. Boston, MA: MIT Press. [ Google Scholar ]
- Versace R., Vallet G. T., Brunel L., Riou B., Lesourd M., Labeye E. (2014). ACT-IN: an integrated view of memory mechanisms. J. Cogn. Psychol. 26 280–306. 10.1080/02699931.2017.1387101 [ PubMed ] [ CrossRef ] [ Google Scholar ]
- Whittlesea B. W. A. (1987). Preservation of specific experiences in the representation of general knowledge. Cognition 13 3–17. 10.1037//0278-7393.13.1.3 [ CrossRef ] [ Google Scholar ]
- Wiener N. (1961). Cybernetics: or Control and Communication in the Animal and the Machine , 2nd Edn Cambridge, MA: MIT Press. [ Google Scholar ]
- Wiener N. (1988). The Human Use of Human Beings: Cybernetics and Society (No. 320). Cambridge, MA: Da Capo Press. [ Google Scholar ]

IMAGES
VIDEO
COMMENTS
Finally, we provide suggestions for future research to establish whether muscle memory exists in humans. 2. ... without any underlying loss of myonuclei as was also rightfully addressed by the authors of the original paper in their recent letter‐to‐the‐editor 13 as a response to this "secondary analysis." Based on this, as well as the ...
The concept of "muscle memory by myonuclear permanence" has mainly been based on data attained from rodent experimental models. Whether the postulated mechanism also holds true in humans remains largely ambiguous. Nevertheless, there are several studies in humans that provide evidence to potentially support or contradict (parts of) the muscle ...
Skeletal muscle memory is an exciting phenomenon gaining significant traction across several scientific communities, among exercise practitioners, and the public. Research has demonstrated that skeletal muscle tissue can be "primed" by earlier positive encounters with exercise training that can enhance adaptation to later retraining, even following significant periods of exercise cessation ...
Skeletal muscle memory is an exciting phenomenon gaining significant traction across several scientific communities, among exercise practitioners, and the public. Research has demonstrated that skeletal muscle tissue can be "primed" by earlier positive encounters with exercise training that can enhance adaptation to later retraining, even ...
FIGURE 4 Changes in myonuclear domain size (A), myonuclear content (B) and muscle fibre size (C) in healthy older. adults with a relatively small (<1600 µm; Small group; n = 15) or. large (>1800 ...
Key points: Referring to the muscle memory theory, previously trained muscles acquire strength and volume much faster than naive muscles. Using extreme experimental models such as synergist ablation or steroid administration, previous studies have demonstrated that the number of nuclei increases when a muscle becomes enlarged, which serves as a cellular muscle memory mechanism for the muscle.
The article by Liu et al. (2011) reports that there are all-or-none action potentials in C. elegans muscles. This study from Zhao-Wen Wang's laboratory demonstrates that these action potentials are calcium dependent and occur in spontaneous trains. By recording from mutant animals, Liu and colleagues identified the ion channels that contribute ...
Introduction. Skeletal muscle fibres are some of the largest cells in the body and uniquely multinucleated with more than one hundred myonuclei per mm length of fibre. 1 In order to maximize the distance between neighbouring nuclei, all nuclei within the syncytium are evenly positioned, adjacent to the plasma membrane. 2 More interestingly, skeletal muscle is an extraordinary tissue with the ...
It is unknown if adult human skeletal muscle has an epigenetic memory of earlier encounters with growth. We report, for the first time in humans, genome-wide DNA methylation (850,000 CpGs) and ...
The term cell memory has also been used to describe irreversible programming of cells such as stem cells, and is attributed to epigenetic mechanisms such as histone and DNA modifications other than changes in base sequence (e.g. Alvarez and Margulies, 2014; Li and Zhang, 2014).For example, it has been demonstrated that fibroblasts in culture maintain a specific gene expression pattern ...
The Journal of Physiology publishes research in all areas of physiology and pathophysiology that illustrates new physiological principles or mechanisms. Key points Referring to the muscle memory theory, previously trained muscles acquire strength and volume much faster than naive muscles. ... Research Paper.
Finally, we provide suggestions for future research to establish whether muscle memory exists in humans. ... without any underlying loss of myonuclei as was also rightfully addressed by the authors of the original paper in their recent letter-to-the-editor 103 as a response to this "secondary analysis." Based on this, as well as the other ...
Due to the small and variable effects of the training on relevant parameters, we were unable to draw any conclusions one way or the other related to muscle memory in humans. We have previously suggested that myonuclei acquired from satellite cells during hypertrophy, and subsequently not lost, could serve as a mechanism for muscle memory ( 3, 6 ...
A memory based on an elevated number of myonuclei could be very long lasting since muscle is a permanent tissue. In humans, the turnover of cells has been studied by utilizing the peak in 14 C availability after atmospheric post‐war testing of nuclear bombs. For skeletal muscle, the half‐life was estimated to be 15 years (Spalding et al. 2005).
Muscle memory. In the movie 'Burn after reading', George Clooney sings the praises of 'muscle memory'- the concept that muscles have excitable circuitry capable of directing complex behaviour on their own. Sure enough, when the moment comes his muscles remember to rapidly draw a gun and shoot a man who inadvertently surprises him.
Stanford neuroscientists observe memory formation in real time. Watch on. In their new study, published July 8, 2022 in Neuron, the researchers trained mice to use their paws to reach food pellets through a small slot. Using genetic wizardry developed by the lab of Liqun Luo, a Wu Tsai Neurosciences Institute colleague in the Department of ...
A study led by researchers at Keele University has shown for the first time that human muscles possess a 'memory' of earlier growth -- at the DNA level. Periods of skeletal muscle growth are ...
Memory is a process in which information is encoded, stored, and retrieved. For vertebrates, the modern view has been that it occurs only in the brain. This review describes a cellular memory in skeletal muscle in which hypertrophy is 'remembered' such that a fibre that has previously been large, but subsequently lost its mass, can regain mass ...
Here, we have discussed the recent progress in artificial muscle research involving different driving mechanisms, including shape memory materials, pneumatic and hydraulic microactuators, DEs ...
Such myonuclear permanence has been suggested to constitute a mechanism allowing the muscle fibre to (re)grow more efficiently during retraining, a phenomenon referred to as "muscle memory.". The concept of "muscle memory by myonuclear permanence" has mainly been based on data attained from rodent experimental models.
Abstract. "Muscle memory" refers to the human body's muscles have memory effects. After the same action is repeated many times, the muscles will form conditioned reflexes. With the development of screen technology, after more and more physical buttons are replaced with screen operations, the structure of the machine no longer follows its ...
Clinical Body Memory (CBM) Mechanisms. The key hypothesis discussed here is that stored bodily experiences of the past and associated emotions (blue boxes) can contribute to the development of Clinical Body Memory (CBM) mechanisms including trauma, pain, dissociation and general somatic symptoms (red box) via neuronal and cognitive mechanisms that mediate their storage and retrieval (yellow box).
Leave No Context Behind: Efficient Infinite Context Transformers with Infini-attention. This work introduces an efficient method to scale Transformer-based Large Language Models (LLMs) to infinitely long inputs with bounded memory and computation. A key component in our proposed approach is a new attention technique dubbed Infini-attention.
The paper introduces Infini-attention, a technique that configures language models in a way that extends their "context window" while keeping memory and compute requirements constant.
In contrast, "memory" now is used to refer to storage of information in general, including in DNA, digital information storage, and neuro-chemical processes. Today, science has moved far beyond a popular understanding of memory as fixed, subjective, and personal. In the extended definition, it is simply the capacity to store and retrieve ...