- Open access
- Published: 26 February 2019

Stem cells: past, present, and future
- Wojciech Zakrzewski 1 ,
- Maciej Dobrzyński 2 ,
- Maria Szymonowicz 1 &
- Zbigniew Rybak 1
Stem Cell Research & Therapy volume 10 , Article number: 68 ( 2019 ) Cite this article
555k Accesses
822 Citations
54 Altmetric
Metrics details
In recent years, stem cell therapy has become a very promising and advanced scientific research topic. The development of treatment methods has evoked great expectations. This paper is a review focused on the discovery of different stem cells and the potential therapies based on these cells. The genesis of stem cells is followed by laboratory steps of controlled stem cell culturing and derivation. Quality control and teratoma formation assays are important procedures in assessing the properties of the stem cells tested. Derivation methods and the utilization of culturing media are crucial to set proper environmental conditions for controlled differentiation. Among many types of stem tissue applications, the use of graphene scaffolds and the potential of extracellular vesicle-based therapies require attention due to their versatility. The review is summarized by challenges that stem cell therapy must overcome to be accepted worldwide. A wide variety of possibilities makes this cutting edge therapy a turning point in modern medicine, providing hope for untreatable diseases.
Stem cell classification
Stem cells are unspecialized cells of the human body. They are able to differentiate into any cell of an organism and have the ability of self-renewal. Stem cells exist both in embryos and adult cells. There are several steps of specialization. Developmental potency is reduced with each step, which means that a unipotent stem cell is not able to differentiate into as many types of cells as a pluripotent one. This chapter will focus on stem cell classification to make it easier for the reader to comprehend the following chapters.
Totipotent stem cells are able to divide and differentiate into cells of the whole organism. Totipotency has the highest differentiation potential and allows cells to form both embryo and extra-embryonic structures. One example of a totipotent cell is a zygote, which is formed after a sperm fertilizes an egg. These cells can later develop either into any of the three germ layers or form a placenta. After approximately 4 days, the blastocyst’s inner cell mass becomes pluripotent. This structure is the source of pluripotent cells.
Pluripotent stem cells (PSCs) form cells of all germ layers but not extraembryonic structures, such as the placenta. Embryonic stem cells (ESCs) are an example. ESCs are derived from the inner cell mass of preimplantation embryos. Another example is induced pluripotent stem cells (iPSCs) derived from the epiblast layer of implanted embryos. Their pluripotency is a continuum, starting from completely pluripotent cells such as ESCs and iPSCs and ending on representatives with less potency—multi-, oligo- or unipotent cells. One of the methods to assess their activity and spectrum is the teratoma formation assay. iPSCs are artificially generated from somatic cells, and they function similarly to PSCs. Their culturing and utilization are very promising for present and future regenerative medicine.
Multipotent stem cells have a narrower spectrum of differentiation than PSCs, but they can specialize in discrete cells of specific cell lineages. One example is a haematopoietic stem cell, which can develop into several types of blood cells. After differentiation, a haematopoietic stem cell becomes an oligopotent cell. Its differentiation abilities are then restricted to cells of its lineage. However, some multipotent cells are capable of conversion into unrelated cell types, which suggests naming them pluripotent cells.
Oligopotent stem cells can differentiate into several cell types. A myeloid stem cell is an example that can divide into white blood cells but not red blood cells.
Unipotent stem cells are characterized by the narrowest differentiation capabilities and a special property of dividing repeatedly. Their latter feature makes them a promising candidate for therapeutic use in regenerative medicine. These cells are only able to form one cell type, e.g. dermatocytes.
Stem cell biology
A blastocyst is formed after the fusion of sperm and ovum fertilization. Its inner wall is lined with short-lived stem cells, namely, embryonic stem cells. Blastocysts are composed of two distinct cell types: the inner cell mass (ICM), which develops into epiblasts and induces the development of a foetus, and the trophectoderm (TE). Blastocysts are responsible for the regulation of the ICM microenvironment. The TE continues to develop and forms the extraembryonic support structures needed for the successful origin of the embryo, such as the placenta. As the TE begins to form a specialized support structure, the ICM cells remain undifferentiated, fully pluripotent and proliferative [ 1 ]. The pluripotency of stem cells allows them to form any cell of the organism. Human embryonic stem cells (hESCs) are derived from the ICM. During the process of embryogenesis, cells form aggregations called germ layers: endoderm, mesoderm and ectoderm (Fig. 1 ), each eventually giving rise to differentiated cells and tissues of the foetus and, later on, the adult organism [ 2 ]. After hESCs differentiate into one of the germ layers, they become multipotent stem cells, whose potency is limited to only the cells of the germ layer. This process is short in human development. After that, pluripotent stem cells occur all over the organism as undifferentiated cells, and their key abilities are proliferation by the formation of the next generation of stem cells and differentiation into specialized cells under certain physiological conditions.
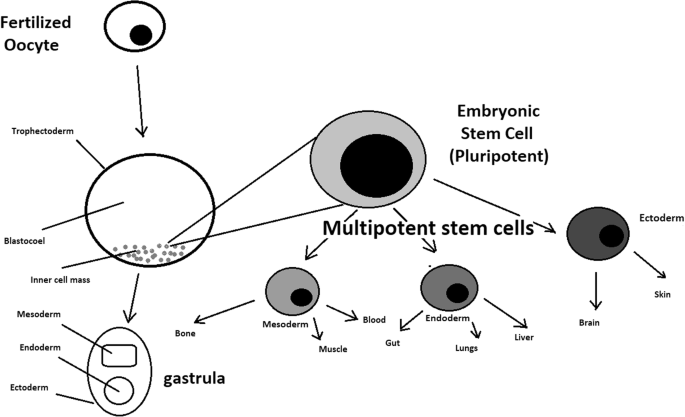
Oocyte development and formation of stem cells: the blastocoel, which is formed from oocytes, consists of embryonic stem cells that later differentiate into mesodermal, ectodermal, or endodermal cells. Blastocoel develops into the gastrula
Signals that influence the stem cell specialization process can be divided into external, such as physical contact between cells or chemical secretion by surrounding tissue, and internal, which are signals controlled by genes in DNA.
Stem cells also act as internal repair systems of the body. The replenishment and formation of new cells are unlimited as long as an organism is alive. Stem cell activity depends on the organ in which they are in; for example, in bone marrow, their division is constant, although in organs such as the pancreas, division only occurs under special physiological conditions.
Stem cell functional division
Whole-body development.
During division, the presence of different stem cells depends on organism development. Somatic stem cell ESCs can be distinguished. Although the derivation of ESCs without separation from the TE is possible, such a combination has growth limits. Because proliferating actions are limited, co-culture of these is usually avoided.
ESCs are derived from the inner cell mass of the blastocyst, which is a stage of pre-implantation embryo ca. 4 days after fertilization. After that, these cells are placed in a culture dish filled with culture medium. Passage is an inefficient but popular process of sub-culturing cells to other dishes. These cells can be described as pluripotent because they are able to eventually differentiate into every cell type in the organism. Since the beginning of their studies, there have been ethical restrictions connected to the medical use of ESCs in therapies. Most embryonic stem cells are developed from eggs that have been fertilized in an in vitro clinic, not from eggs fertilized in vivo.
Somatic or adult stem cells are undifferentiated and found among differentiated cells in the whole body after development. The function of these cells is to enable the healing, growth, and replacement of cells that are lost each day. These cells have a restricted range of differentiation options. Among many types, there are the following:
Mesenchymal stem cells are present in many tissues. In bone marrow, these cells differentiate mainly into the bone, cartilage, and fat cells. As stem cells, they are an exception because they act pluripotently and can specialize in the cells of any germ layer.
Neural cells give rise to nerve cells and their supporting cells—oligodendrocytes and astrocytes.
Haematopoietic stem cells form all kinds of blood cells: red, white, and platelets.
Skin stem cells form, for example, keratinocytes, which form a protective layer of skin.
The proliferation time of somatic stem cells is longer than that of ESCs. It is possible to reprogram adult stem cells back to their pluripotent state. This can be performed by transferring the adult nucleus into the cytoplasm of an oocyte or by fusion with the pluripotent cell. The same technique was used during cloning of the famous Dolly sheep.
hESCs are involved in whole-body development. They can differentiate into pluripotent, totipotent, multipotent, and unipotent cells (Fig. 2 ) [ 2 ].
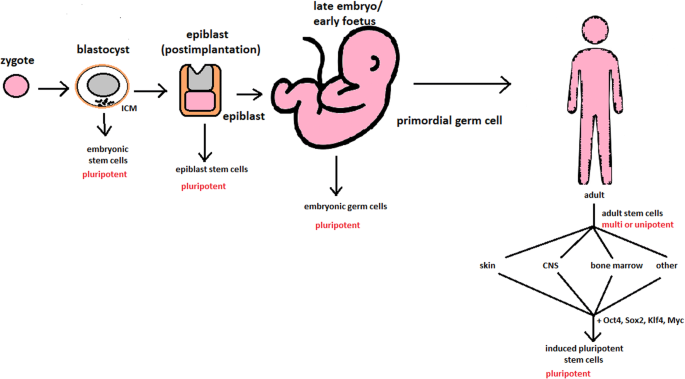
Changes in the potency of stem cells in human body development. Potency ranges from pluripotent cells of the blastocyst to unipotent cells of a specific tissue in a human body such as the skin, CNS, or bone marrow. Reversed pluripotency can be achieved by the formation of induced pluripotent stem cells using either octamer-binding transcription factor (Oct4), sex-determining region Y (Sox2), Kruppel-like factor 4 (Klf4), or the Myc gene
Pluripotent cells can be named totipotent if they can additionally form extraembryonic tissues of the embryo. Multipotent cells are restricted in differentiating to each cell type of given tissue. When tissue contains only one lineage of cells, stem cells that form them are called either called oligo- or unipotent.
iPSC quality control and recognition by morphological differences
The comparability of stem cell lines from different individuals is needed for iPSC lines to be used in therapeutics [ 3 ]. Among critical quality procedures, the following can be distinguished:
Short tandem repeat analysis—This is the comparison of specific loci on the DNA of the samples. It is used in measuring an exact number of repeating units. One unit consists of 2 to 13 nucleotides repeating many times on the DNA strand. A polymerase chain reaction is used to check the lengths of short tandem repeats. The genotyping procedure of source tissue, cells, and iPSC seed and master cell banks is recommended.
Identity analysis—The unintentional switching of lines, resulting in other stem cell line contamination, requires rigorous assay for cell line identification.
Residual vector testing—An appearance of reprogramming vectors integrated into the host genome is hazardous, and testing their presence is a mandatory procedure. It is a commonly used procedure for generating high-quality iPSC lines. An acceptable threshold in high-quality research-grade iPSC line collections is ≤ 1 plasmid copies per 100 cells. During the procedure, 2 different regions, common to all plasmids, should be used as specific targets, such as EBNA and CAG sequences [ 3 ]. To accurately represent the test reactions, a standard curve needs to be prepared in a carrier of gDNA from a well-characterized hPSC line. For calculations of plasmid copies per cell, it is crucial to incorporate internal reference gDNA sequences to allow the quantification of, for example, ribonuclease P (RNaseP) or human telomerase reverse transcriptase (hTERT).
Karyotype—A long-term culture of hESCs can accumulate culture-driven mutations [ 4 ]. Because of that, it is crucial to pay additional attention to genomic integrity. Karyotype tests can be performed by resuscitating representative aliquots and culturing them for 48–72 h before harvesting cells for karyotypic analysis. If abnormalities are found within the first 20 karyotypes, the analysis must be repeated on a fresh sample. When this situation is repeated, the line is evaluated as abnormal. Repeated abnormalities must be recorded. Although karyology is a crucial procedure in stem cell quality control, the single nucleotide polymorphism (SNP) array, discussed later, has approximately 50 times higher resolution.
Viral testing—When assessing the quality of stem cells, all tests for harmful human adventitious agents must be performed (e.g. hepatitis C or human immunodeficiency virus). This procedure must be performed in the case of non-xeno-free culture agents.
Bacteriology—Bacterial or fungal sterility tests can be divided into culture- or broth-based tests. All the procedures must be recommended by pharmacopoeia for the jurisdiction in which the work is performed.
Single nucleotide polymorphism arrays—This procedure is a type of DNA microarray that detects population polymorphisms by enabling the detection of subchromosomal changes and the copy-neutral loss of heterozygosity, as well as an indication of cellular transformation. The SNP assay consists of three components. The first is labelling fragmented nucleic acid sequences with fluorescent dyes. The second is an array that contains immobilized allele-specific oligonucleotide (ASO) probes. The last component detects, records, and eventually interprets the signal.
Flow cytometry—This is a technique that utilizes light to count and profile cells in a heterogeneous fluid mixture. It allows researchers to accurately and rapidly collect data from heterogeneous fluid mixtures with live cells. Cells are passed through a narrow channel one by one. During light illumination, sensors detect light emitted or refracted from the cells. The last step is data analysis, compilation and integration into a comprehensive picture of the sample.
Phenotypic pluripotency assays—Recognizing undifferentiated cells is crucial in successful stem cell therapy. Among other characteristics, stem cells appear to have a distinct morphology with a high nucleus to cytoplasm ratio and a prominent nucleolus. Cells appear to be flat with defined borders, in contrast to differentiating colonies, which appear as loosely located cells with rough borders [ 5 ]. It is important that images of ideal and poor quality colonies for each cell line are kept in laboratories, so whenever there is doubt about the quality of culture, it can always be checked according to the representative image. Embryoid body formation or directed differentiation of monolayer cultures to produce cell types representative of all three embryonic germ layers must be performed. It is important to note that colonies cultured under different conditions may have different morphologies [ 6 ].
Histone modification and DNA methylation—Quality control can be achieved by using epigenetic analysis tools such as histone modification or DNA methylation. When stem cells differentiate, the methylation process silences pluripotency genes, which reduces differentiation potential, although other genes may undergo demethylation to become expressed [ 7 ]. It is important to emphasize that stem cell identity, together with its morphological characteristics, is also related to its epigenetic profile [ 8 , 9 ]. According to Brindley [ 10 ], there is a relationship between epigenetic changes, pluripotency, and cell expansion conditions, which emphasizes that unmethylated regions appear to be serum-dependent.
hESC derivation and media
hESCs can be derived using a variety of methods, from classic culturing to laser-assisted methodologies or microsurgery [ 11 ]. hESC differentiation must be specified to avoid teratoma formation (see Fig. 3 ).
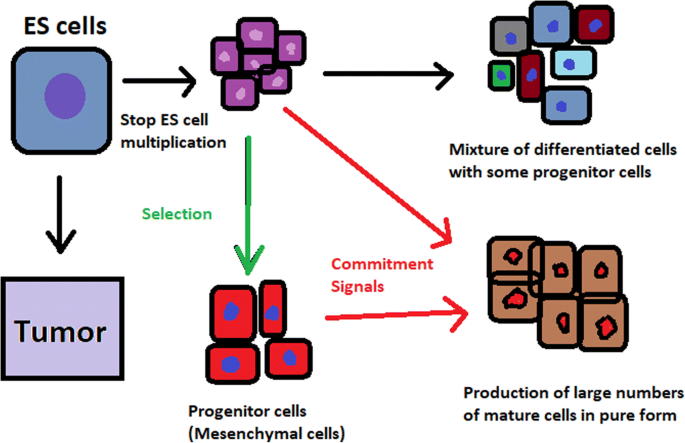
Spontaneous differentiation of hESCs causes the formation of a heterogeneous cell population. There is a different result, however, when commitment signals (in forms of soluble factors and culture conditions) are applied and enable the selection of progenitor cells
hESCs spontaneously differentiate into embryonic bodies (EBs) [ 12 ]. EBs can be studied instead of embryos or animals to predict their effects on early human development. There are many different methods for acquiring EBs, such as bioreactor culture [ 13 ], hanging drop culture [ 12 ], or microwell technology [ 14 , 15 ]. These methods allow specific precursors to form in vitro [ 16 ].
The essential part of these culturing procedures is a separation of inner cell mass to culture future hESCs (Fig. 4 ) [ 17 ]. Rosowski et al. [ 18 ] emphasizes that particular attention must be taken in controlling spontaneous differentiation. When the colony reaches the appropriate size, cells must be separated. The occurrence of pluripotent cells lasts for 1–2 days. Because the classical utilization of hESCs caused ethical concerns about gastrulas used during procedures, Chung et al. [ 19 ] found out that it is also possible to obtain hESCs from four cell embryos, leaving a higher probability of embryo survival. Additionally, Zhang et al. [ 20 ] used only in vitro fertilization growth-arrested cells.
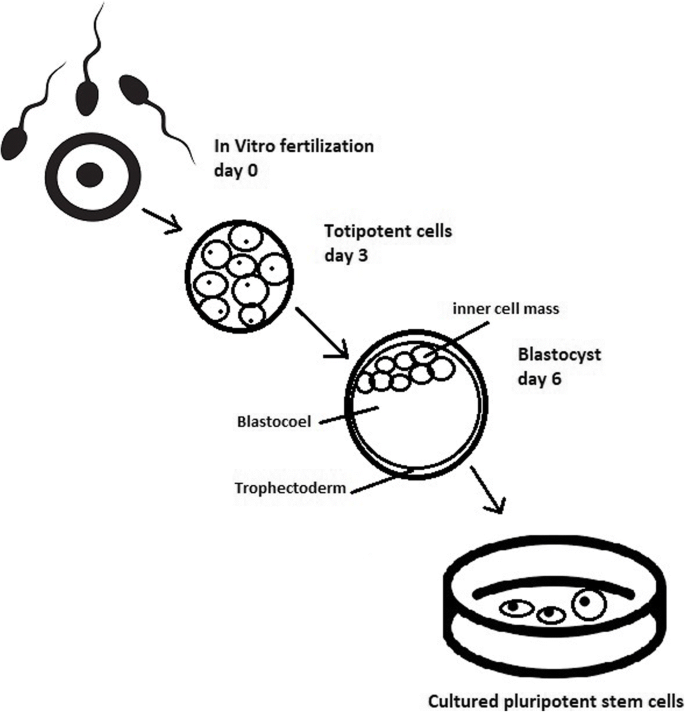
Culturing of pluripotent stem cells in vitro. Three days after fertilization, totipotent cells are formed. Blastocysts with ICM are formed on the sixth day after fertilization. Pluripotent stem cells from ICM can then be successfully transmitted on a dish
Cell passaging is used to form smaller clusters of cells on a new culture surface [ 21 ]. There are four important passaging procedures.
Enzymatic dissociation is a cutting action of enzymes on proteins and adhesion domains that bind the colony. It is a gentler method than the manual passage. It is crucial to not leave hESCs alone after passaging. Solitary cells are more sensitive and can easily undergo cell death; collagenase type IV is an example [ 22 , 23 ].
Manual passage , on the other hand, focuses on using cell scratchers. The selection of certain cells is not necessary. This should be done in the early stages of cell line derivation [ 24 ].
Trypsin utilization allows a healthy, automated hESC passage. Good Manufacturing Practice (GMP)-grade recombinant trypsin is widely available in this procedure [ 24 ]. However, there is a risk of decreasing the pluripotency and viability of stem cells [ 25 ]. Trypsin utilization can be halted with an inhibitor of the protein rho-associated protein kinase (ROCK) [ 26 ].
Ethylenediaminetetraacetic acid ( EDTA ) indirectly suppresses cell-to-cell connections by chelating divalent cations. Their suppression promotes cell dissociation [ 27 ].
Stem cells require a mixture of growth factors and nutrients to differentiate and develop. The medium should be changed each day.
Traditional culture methods used for hESCs are mouse embryonic fibroblasts (MEFs) as a feeder layer and bovine serum [ 28 ] as a medium. Martin et al. [ 29 ] demonstrated that hESCs cultured in the presence of animal products express the non-human sialic acid, N -glycolylneuraminic acid (NeuGc). Feeder layers prevent uncontrolled proliferation with factors such as leukaemia inhibitory factor (LIF) [ 30 ].
First feeder layer-free culture can be supplemented with serum replacement, combined with laminin [ 31 ]. This causes stable karyotypes of stem cells and pluripotency lasting for over a year.
Initial culturing media can be serum (e.g. foetal calf serum FCS), artificial replacement such as synthetic serum substitute (SSS), knockout serum replacement (KOSR), or StemPro [ 32 ]. The simplest culture medium contains only eight essential elements: DMEM/F12 medium, selenium, NaHCO 3, l -ascorbic acid, transferrin, insulin, TGFβ1, and FGF2 [ 33 ]. It is not yet fully known whether culture systems developed for hESCs can be allowed without adaptation in iPSC cultures.
Turning point in stem cell therapy
The turning point in stem cell therapy appeared in 2006, when scientists Shinya Yamanaka, together with Kazutoshi Takahashi, discovered that it is possible to reprogram multipotent adult stem cells to the pluripotent state. This process avoided endangering the foetus’ life in the process. Retrovirus-mediated transduction of mouse fibroblasts with four transcription factors (Oct-3/4, Sox2, KLF4, and c-Myc) [ 34 ] that are mainly expressed in embryonic stem cells could induce the fibroblasts to become pluripotent (Fig. 5 ) [ 35 ]. This new form of stem cells was named iPSCs. One year later, the experiment also succeeded with human cells [ 36 ]. After this success, the method opened a new field in stem cell research with a generation of iPSC lines that can be customized and biocompatible with the patient. Recently, studies have focused on reducing carcinogenesis and improving the conduction system.
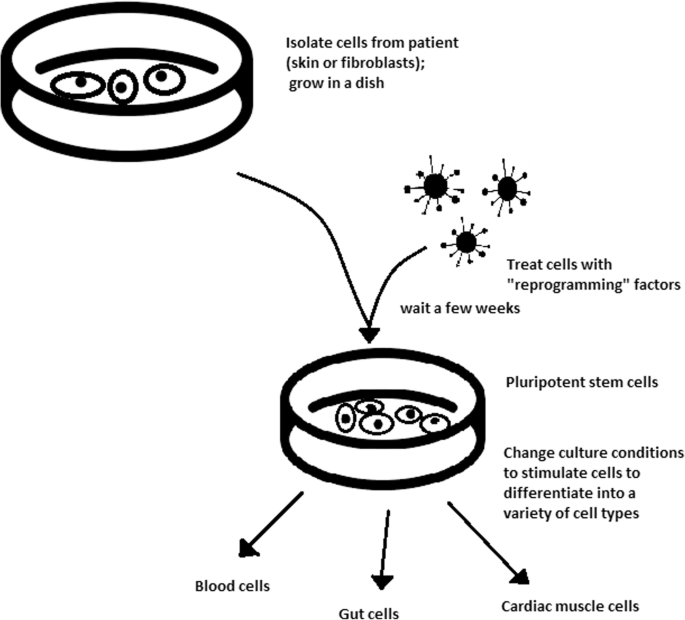
Retroviral-mediated transduction induces pluripotency in isolated patient somatic cells. Target cells lose their role as somatic cells and, once again, become pluripotent and can differentiate into any cell type of human body
The turning point was influenced by former discoveries that happened in 1962 and 1987.
The former discovery was about scientist John Gurdon successfully cloning frogs by transferring a nucleus from a frog’s somatic cells into an oocyte. This caused a complete reversion of somatic cell development [ 37 ]. The results of his experiment became an immense discovery since it was previously believed that cell differentiation is a one-way street only, but his experiment suggested the opposite and demonstrated that it is even possible for a somatic cell to again acquire pluripotency [ 38 ].
The latter was a discovery made by Davis R.L. that focused on fibroblast DNA subtraction. Three genes were found that originally appeared in myoblasts. The enforced expression of only one of the genes, named myogenic differentiation 1 (Myod1), caused the conversion of fibroblasts into myoblasts, showing that reprogramming cells is possible, and it can even be used to transform cells from one lineage to another [ 39 ].
Although pluripotency can occur naturally only in embryonic stem cells, it is possible to induce terminally differentiated cells to become pluripotent again. The process of direct reprogramming converts differentiated somatic cells into iPSC lines that can form all cell types of an organism. Reprogramming focuses on the expression of oncogenes such as Myc and Klf4 (Kruppel-like factor 4). This process is enhanced by a downregulation of genes promoting genome stability, such as p53. Additionally, cell reprogramming involves histone alteration. All these processes can cause potential mutagenic risk and later lead to an increased number of mutations. Quinlan et al. [ 40 ] checked fully pluripotent mouse iPSCs using whole genome DNA sequencing and structural variation (SV) detection algorithms. Based on those studies, it was confirmed that although there were single mutations in the non-genetic region, there were non-retrotransposon insertions. This led to the conclusion that current reprogramming methods can produce fully pluripotent iPSCs without severe genomic alterations.
During the course of development from pluripotent hESCs to differentiated somatic cells, crucial changes appear in the epigenetic structure of these cells. There is a restriction or permission of the transcription of genes relevant to each cell type. When somatic cells are being reprogrammed using transcription factors, all the epigenetic architecture has to be reconditioned to achieve iPSCs with pluripotency [ 41 ]. However, cells of each tissue undergo specific somatic genomic methylation. This influences transcription, which can further cause alterations in induced pluripotency [ 42 ].
Source of iPSCs
Because pluripotent cells can propagate indefinitely and differentiate into any kind of cell, they can be an unlimited source, either for replacing lost or diseased tissues. iPSCs bypass the need for embryos in stem cell therapy. Because they are made from the patient’s own cells, they are autologous and no longer generate any risk of immune rejection.
At first, fibroblasts were used as a source of iPSCs. Because a biopsy was needed to achieve these types of cells, the technique underwent further research. Researchers investigated whether more accessible cells could be used in the method. Further, other cells were used in the process: peripheral blood cells, keratinocytes, and renal epithelial cells found in urine. An alternative strategy to stem cell transplantation can be stimulating a patient’s endogenous stem cells to divide or differentiate, occurring naturally when skin wounds are healing. In 2008, pancreatic exocrine cells were shown to be reprogrammed to functional, insulin-producing beta cells [ 43 ].
The best stem cell source appears to be the fibroblasts, which is more tempting in the case of logistics since its stimulation can be fast and better controlled [ 44 ].
- Teratoma formation assay
The self-renewal and differentiation capabilities of iPSCs have gained significant interest and attention in regenerative medicine sciences. To study their abilities, a quality-control assay is needed, of which one of the most important is the teratoma formation assay. Teratomas are benign tumours. Teratomas are capable of rapid growth in vivo and are characteristic because of their ability to develop into tissues of all three germ layers simultaneously. Because of the high pluripotency of teratomas, this formation assay is considered an assessment of iPSC’s abilities [ 45 ].
Teratoma formation rate, for instance, was observed to be elevated in human iPSCs compared to that in hESCs [ 46 ]. This difference may be connected to different differentiation methods and cell origins. Most commonly, the teratoma assay involves an injection of examined iPSCs subcutaneously or under the testis or kidney capsule in mice, which are immune-deficient [ 47 ]. After injection, an immature but recognizable tissue can be observed, such as the kidney tubules, bone, cartilage, or neuroepithelium [ 30 ]. The injection site may have an impact on the efficiency of teratoma formation [ 48 ].
There are three groups of markers used in this assay to differentiate the cells of germ layers. For endodermal tissue, there is insulin/C-peptide and alpha-1 antitrypsin [ 49 ]. For the mesoderm, derivatives can be used, e.g. cartilage matrix protein for the bone and alcian blue for the cartilage. As ectodermal markers, class III B botulin or keratin can be used for keratinocytes.
Teratoma formation assays are considered the gold standard for demonstrating the pluripotency of human iPSCs, demonstrating their possibilities under physiological conditions. Due to their actual tissue formation, they could be used for the characterization of many cell lineages [ 50 ].
Directed differentiation
To be useful in therapy, stem cells must be converted into desired cell types as necessary or else the whole regenerative medicine process will be pointless. Differentiation of ESCs is crucial because undifferentiated ESCs can cause teratoma formation in vivo. Understanding and using signalling pathways for differentiation is an important method in successful regenerative medicine. In directed differentiation, it is likely to mimic signals that are received by cells when they undergo successive stages of development [ 51 ]. The extracellular microenvironment plays a significant role in controlling cell behaviour. By manipulating the culture conditions, it is possible to restrict specific differentiation pathways and generate cultures that are enriched in certain precursors in vitro. However, achieving a similar effect in vivo is challenging. It is crucial to develop culture conditions that will allow the promotion of homogenous and enhanced differentiation of ESCs into functional and desired tissues.
Regarding the self-renewal of embryonic stem cells, Hwang et al. [ 52 ] noted that the ideal culture method for hESC-based cell and tissue therapy would be a defined culture free of either the feeder layer or animal components. This is because cell and tissue therapy requires the maintenance of large quantities of undifferentiated hESCs, which does not make feeder cells suitable for such tasks.
Most directed differentiation protocols are formed to mimic the development of an inner cell mass during gastrulation. During this process, pluripotent stem cells differentiate into ectodermal, mesodermal, or endodermal progenitors. Mall molecules or growth factors induce the conversion of stem cells into appropriate progenitor cells, which will later give rise to the desired cell type. There is a variety of signal intensities and molecular families that may affect the establishment of germ layers in vivo, such as fibroblast growth factors (FGFs) [ 53 ]; the Wnt family [ 54 ] or superfamily of transforming growth factors—β(TGFβ); and bone morphogenic proteins (BMP) [ 55 ]. Each candidate factor must be tested on various concentrations and additionally applied to various durations because the precise concentrations and times during which developing cells in embryos are influenced during differentiation are unknown. For instance, molecular antagonists of endogenous BMP and Wnt signalling can be used for ESC formation of ectoderm [ 56 ]. However, transient Wnt and lower concentrations of the TGFβ family trigger mesodermal differentiation [ 57 ]. Regarding endoderm formation, a higher activin A concentration may be required [ 58 , 59 ].
There are numerous protocols about the methods of forming progenitors of cells of each of germ layers, such as cardiomyocytes [ 60 ], hepatocytes [ 61 ], renal cells [ 62 ], lung cells [ 63 , 64 ], motor neurons [ 65 ], intestinal cells [ 66 ], or chondrocytes [ 67 ].
Directed differentiation of either iPSCs or ESCs into, e.g. hepatocytes, could influence and develop the study of the molecular mechanisms in human liver development. In addition, it could also provide the possibility to form exogenous hepatocytes for drug toxicity testing [ 68 ].
Levels of concentration and duration of action with a specific signalling molecule can cause a variety of factors. Unfortunately, for now, a high cost of recombinant factors is likely to limit their use on a larger scale in medicine. The more promising technique focuses on the use of small molecules. These can be used for either activating or deactivating specific signalling pathways. They enhance reprogramming efficiency by creating cells that are compatible with the desired type of tissue. It is a cheaper and non-immunogenic method.
One of the successful examples of small-molecule cell therapies is antagonists and agonists of the Hedgehog pathway. They show to be very useful in motor neuron regeneration [ 69 ]. Endogenous small molecules with their function in embryonic development can also be used in in vitro methods to induce the differentiation of cells; for example, retinoic acid, which is responsible for patterning the nervous system in vivo [ 70 ], surprisingly induced retinal cell formation when the laboratory procedure involved hESCs [ 71 ].
The efficacy of differentiation factors depends on functional maturity, efficiency, and, finally, introducing produced cells to their in vivo equivalent. Topography, shear stress, and substrate rigidity are factors influencing the phenotype of future cells [ 72 ].
The control of biophysical and biochemical signals, the biophysical environment, and a proper guide of hESC differentiation are important factors in appropriately cultured stem cells.
Stem cell utilization and their manufacturing standards and culture systems
The European Medicines Agency and the Food and Drug Administration have set Good Manufacturing Practice (GMP) guidelines for safe and appropriate stem cell transplantation. In the past, protocols used for stem cell transplantation required animal-derived products [ 73 ].
The risk of introducing animal antigens or pathogens caused a restriction in their use. Due to such limitations, the technique required an obvious update [ 74 ]. Now, it is essential to use xeno-free equivalents when establishing cell lines that are derived from fresh embryos and cultured from human feeder cell lines [ 75 ]. In this method, it is crucial to replace any non-human materials with xeno-free equivalents [ 76 ].
NutriStem with LN-511, TeSR2 with human recombinant laminin (LN-511), and RegES with human foreskin fibroblasts (HFFs) are commonly used xeno-free culture systems [ 33 ]. There are many organizations and international initiatives, such as the National Stem Cell Bank, that provide stem cell lines for treatment or medical research [ 77 ].
Stem cell use in medicine
Stem cells have great potential to become one of the most important aspects of medicine. In addition to the fact that they play a large role in developing restorative medicine, their study reveals much information about the complex events that happen during human development.
The difference between a stem cell and a differentiated cell is reflected in the cells’ DNA. In the former cell, DNA is arranged loosely with working genes. When signals enter the cell and the differentiation process begins, genes that are no longer needed are shut down, but genes required for the specialized function will remain active. This process can be reversed, and it is known that such pluripotency can be achieved by interaction in gene sequences. Takahashi and Yamanaka [ 78 ] and Loh et al. [ 79 ] discovered that octamer-binding transcription factor 3 and 4 (Oct3/4), sex determining region Y (SRY)-box 2 and Nanog genes function as core transcription factors in maintaining pluripotency. Among them, Oct3/4 and Sox2 are essential for the generation of iPSCs.
Many serious medical conditions, such as birth defects or cancer, are caused by improper differentiation or cell division. Currently, several stem cell therapies are possible, among which are treatments for spinal cord injury, heart failure [ 80 ], retinal and macular degeneration [ 81 ], tendon ruptures, and diabetes type 1 [ 82 ]. Stem cell research can further help in better understanding stem cell physiology. This may result in finding new ways of treating currently incurable diseases.
Haematopoietic stem cell transplantation
Haematopoietic stem cells are important because they are by far the most thoroughly characterized tissue-specific stem cell; after all, they have been experimentally studied for more than 50 years. These stem cells appear to provide an accurate paradigm model system to study tissue-specific stem cells, and they have potential in regenerative medicine.
Multipotent haematopoietic stem cell (HSC) transplantation is currently the most popular stem cell therapy. Target cells are usually derived from the bone marrow, peripheral blood, or umbilical cord blood [ 83 ]. The procedure can be autologous (when the patient’s own cells are used), allogenic (when the stem cell comes from a donor), or syngeneic (from an identical twin). HSCs are responsible for the generation of all functional haematopoietic lineages in blood, including erythrocytes, leukocytes, and platelets. HSC transplantation solves problems that are caused by inappropriate functioning of the haematopoietic system, which includes diseases such as leukaemia and anaemia. However, when conventional sources of HSC are taken into consideration, there are some important limitations. First, there is a limited number of transplantable cells, and an efficient way of gathering them has not yet been found. There is also a problem with finding a fitting antigen-matched donor for transplantation, and viral contamination or any immunoreactions also cause a reduction in efficiency in conventional HSC transplantations. Haematopoietic transplantation should be reserved for patients with life-threatening diseases because it has a multifactorial character and can be a dangerous procedure. iPSC use is crucial in this procedure. The use of a patient’s own unspecialized somatic cells as stem cells provides the greatest immunological compatibility and significantly increases the success of the procedure.
Stem cells as a target for pharmacological testing
Stem cells can be used in new drug tests. Each experiment on living tissue can be performed safely on specific differentiated cells from pluripotent cells. If any undesirable effect appears, drug formulas can be changed until they reach a sufficient level of effectiveness. The drug can enter the pharmacological market without harming any live testers. However, to test the drugs properly, the conditions must be equal when comparing the effects of two drugs. To achieve this goal, researchers need to gain full control of the differentiation process to generate pure populations of differentiated cells.
Stem cells as an alternative for arthroplasty
One of the biggest fears of professional sportsmen is getting an injury, which most often signifies the end of their professional career. This applies especially to tendon injuries, which, due to current treatment options focusing either on conservative or surgical treatment, often do not provide acceptable outcomes. Problems with the tendons start with their regeneration capabilities. Instead of functionally regenerating after an injury, tendons merely heal by forming scar tissues that lack the functionality of healthy tissues. Factors that may cause this failed healing response include hypervascularization, deposition of calcific materials, pain, or swelling [ 84 ].
Additionally, in addition to problems with tendons, there is a high probability of acquiring a pathological condition of joints called osteoarthritis (OA) [ 85 ]. OA is common due to the avascular nature of articular cartilage and its low regenerative capabilities [ 86 ]. Although arthroplasty is currently a common procedure in treating OA, it is not ideal for younger patients because they can outlive the implant and will require several surgical procedures in the future. These are situations where stem cell therapy can help by stopping the onset of OA [ 87 ]. However, these procedures are not well developed, and the long-term maintenance of hyaline cartilage requires further research.
Osteonecrosis of the femoral hip (ONFH) is a refractory disease associated with the collapse of the femoral head and risk of hip arthroplasty in younger populations [ 88 ]. Although total hip arthroplasty (THA) is clinically successful, it is not ideal for young patients, mostly due to the limited lifetime of the prosthesis. An increasing number of clinical studies have evaluated the therapeutic effect of stem cells on ONFH. Most of the authors demonstrated positive outcomes, with reduced pain, improved function, or avoidance of THA [ 89 , 90 , 91 ].
Rejuvenation by cell programming
Ageing is a reversible epigenetic process. The first cell rejuvenation study was published in 2011 [ 92 ]. Cells from aged individuals have different transcriptional signatures, high levels of oxidative stress, dysfunctional mitochondria, and shorter telomeres than in young cells [ 93 ]. There is a hypothesis that when human or mouse adult somatic cells are reprogrammed to iPSCs, their epigenetic age is virtually reset to zero [ 94 ]. This was based on an epigenetic model, which explains that at the time of fertilization, all marks of parenteral ageing are erased from the zygote’s genome and its ageing clock is reset to zero [ 95 ].
In their study, Ocampo et al. [ 96 ] used Oct4, Sox2, Klf4, and C-myc genes (OSKM genes) and affected pancreas and skeletal muscle cells, which have poor regenerative capacity. Their procedure revealed that these genes can also be used for effective regenerative treatment [ 97 ]. The main challenge of their method was the need to employ an approach that does not use transgenic animals and does not require an indefinitely long application. The first clinical approach would be preventive, focused on stopping or slowing the ageing rate. Later, progressive rejuvenation of old individuals can be attempted. In the future, this method may raise some ethical issues, such as overpopulation, leading to lower availability of food and energy.
For now, it is important to learn how to implement cell reprogramming technology in non-transgenic elder animals and humans to erase marks of ageing without removing the epigenetic marks of cell identity.
Cell-based therapies
Stem cells can be induced to become a specific cell type that is required to repair damaged or destroyed tissues (Fig. 6 ). Currently, when the need for transplantable tissues and organs outweighs the possible supply, stem cells appear to be a perfect solution for the problem. The most common conditions that benefit from such therapy are macular degenerations [ 98 ], strokes [ 99 ], osteoarthritis [ 89 , 90 ], neurodegenerative diseases, and diabetes [ 100 ]. Due to this technique, it can become possible to generate healthy heart muscle cells and later transplant them to patients with heart disease.
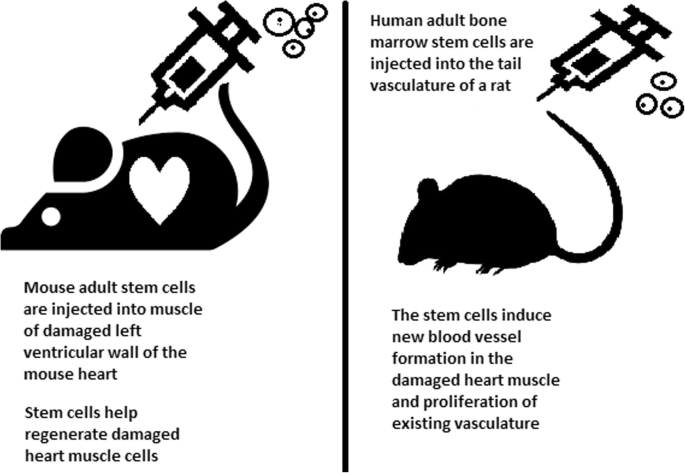
Stem cell experiments on animals. These experiments are one of the many procedures that proved stem cells to be a crucial factor in future regenerative medicine
In the case of type 1 diabetes, insulin-producing cells in the pancreas are destroyed due to an autoimmunological reaction. As an alternative to transplantation therapy, it can be possible to induce stem cells to differentiate into insulin-producing cells [ 101 ].
Stem cells and tissue banks
iPS cells with their theoretically unlimited propagation and differentiation abilities are attractive for the present and future sciences. They can be stored in a tissue bank to be an essential source of human tissue used for medical examination. The problem with conventional differentiated tissue cells held in the laboratory is that their propagation features diminish after time. This does not occur in iPSCs.
The umbilical cord is known to be rich in mesenchymal stem cells. Due to its cryopreservation immediately after birth, its stem cells can be successfully stored and used in therapies to prevent the future life-threatening diseases of a given patient.
Stem cells of human exfoliated deciduous teeth (SHED) found in exfoliated deciduous teeth has the ability to develop into more types of body tissues than other stem cells [ 102 ] (Table 1 ). Techniques of their collection, isolation, and storage are simple and non-invasive. Among the advantages of banking, SHED cells are:
Guaranteed donor-match autologous transplant that causes no immune reaction and rejection of cells [ 103 ]
Simple and painless for both child and parent
Less than one third of the cost of cord blood storage
Not subject to the same ethical concerns as embryonic stem cells [ 104 ]
In contrast to cord blood stem cells, SHED cells are able to regenerate into solid tissues such as connective, neural, dental, or bone tissue [ 105 , 106 ]
SHED can be useful for close relatives of the donor
Fertility diseases
In 2011, two researchers, Katsuhiko Hayashi et al. [ 107 ], showed in an experiment on mice that it is possible to form sperm from iPSCs. They succeeded in delivering healthy and fertile pups in infertile mice. The experiment was also successful for female mice, where iPSCs formed fully functional eggs .
Young adults at risk of losing their spermatogonial stem cells (SSC), mostly cancer patients, are the main target group that can benefit from testicular tissue cryopreservation and autotransplantation. Effective freezing methods for adult and pre-pubertal testicular tissue are available [ 108 ].
Qiuwan et al. [ 109 ] provided important evidence that human amniotic epithelial cell (hAEC) transplantation could effectively improve ovarian function by inhibiting cell apoptosis and reducing inflammation in injured ovarian tissue of mice, and it could be a promising strategy for the management of premature ovarian failure or insufficiency in female cancer survivors.
For now, reaching successful infertility treatments in humans appears to be only a matter of time, but there are several challenges to overcome. First, the process needs to have high efficiency; second, the chances of forming tumours instead of eggs or sperm must be maximally reduced. The last barrier is how to mature human sperm and eggs in the lab without transplanting them to in vivo conditions, which could cause either a tumour risk or an invasive procedure.
Therapy for incurable neurodegenerative diseases
Thanks to stem cell therapy, it is possible not only to delay the progression of incurable neurodegenerative diseases such as Parkinson’s disease, Alzheimer’s disease (AD), and Huntington disease, but also, most importantly, to remove the source of the problem. In neuroscience, the discovery of neural stem cells (NSCs) has nullified the previous idea that adult CNS were not capable of neurogenesis [ 110 , 111 ]. Neural stem cells are capable of improving cognitive function in preclinical rodent models of AD [ 112 , 113 , 114 ]. Awe et al. [ 115 ] clinically derived relevant human iPSCs from skin punch biopsies to develop a neural stem cell-based approach for treating AD. Neuronal degeneration in Parkinson’s disease (PD) is focal, and dopaminergic neurons can be efficiently generated from hESCs. PD is an ideal disease for iPSC-based cell therapy [ 116 ]. However, this therapy is still in an experimental phase ( https://www.ncbi.nlm.nih.gov/pmc/articles/PMC4539501 /). Brain tissue from aborted foetuses was used on patients with Parkinson’s disease [ 117 ]. Although the results were not uniform, they showed that therapies with pure stem cells are an important and achievable therapy.
Stem cell use in dentistry
Teeth represent a very challenging material for regenerative medicine. They are difficult to recreate because of their function in aspects such as articulation, mastication, or aesthetics due to their complicated structure. Currently, there is a chance for stem cells to become more widely used than synthetic materials. Teeth have a large advantage of being the most natural and non-invasive source of stem cells.
For now, without the use of stem cells, the most common periodontological treatments are either growth factors, grafts, or surgery. For example, there are stem cells in periodontal ligament [ 118 , 119 ], which are capable of differentiating into osteoblasts or cementoblasts, and their functions were also assessed in neural cells [ 120 ]. Tissue engineering is a successful method for treating periodontal diseases. Stem cells of the root apical areas are able to recreate periodontal ligament. One of the possible methods of tissue engineering in periodontology is gene therapy performed using adenoviruses-containing growth factors [ 121 ].
As a result of animal studies, dentin regeneration is an effective process that results in the formation of dentin bridges [ 122 ].
Enamel is more difficult to regenerate than dentin. After the differentiation of ameloblastoma cells into the enamel, the former is destroyed, and reparation is impossible. Medical studies have succeeded in differentiating bone marrow stem cells into ameloblastoma [ 123 ].
Healthy dental tissue has a high amount of regular stem cells, although this number is reduced when tissue is either traumatized or inflamed [ 124 ]. There are several dental stem cell groups that can be isolated (Fig. 7 ).
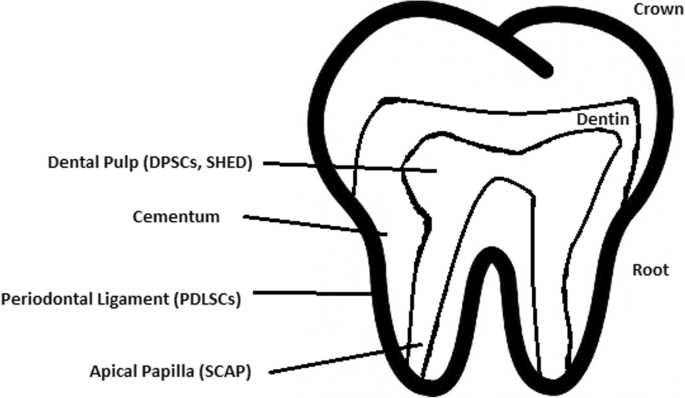
Localization of stem cells in dental tissues. Dental pulp stem cells (DPSCs) and human deciduous teeth stem cells (SHED) are located in the dental pulp. Periodontal ligaments stem cells are located in the periodontal ligament. Apical papilla consists of stem cells from the apical papilla (SCAP)
Dental pulp stem cell (DPSC)
These were the first dental stem cells isolated from the human dental pulp, which were [ 125 ] located inside dental pulp (Table 2 ). They have osteogenic and chondrogenic potential. Mesenchymal stem cells (MSCs) of the dental pulp, when isolated, appear highly clonogenic; they can be isolated from adult tissue (e.g. bone marrow, adipose tissue) and foetal (e.g. umbilical cord) [ 126 ] tissue, and they are able to differentiate densely [ 127 ]. MSCs differentiate into odontoblast-like cells and osteoblasts to form dentin and bone. Their best source locations are the third molars [ 125 ]. DPSCs are the most useful dental source of tissue engineering due to their easy surgical accessibility, cryopreservation possibility, increased production of dentin tissues compared to non-dental stem cells, and their anti-inflammatory abilities. These cells have the potential to be a source for maxillofacial and orthopaedic reconstructions or reconstructions even beyond the oral cavity. DPSCs are able to generate all structures of the developed tooth [ 128 ]. In particular, beneficial results in the use of DPSCs may be achieved when combined with other new therapies, such as periodontal tissue photobiomodulation (laser stimulation), which is an efficient technique in the stimulation of proliferation and differentiation into distinct cell types [ 129 ]. DPSCs can be induced to form neural cells to help treat neurological deficits.
Stem cells of human exfoliated deciduous teeth (SHED) have a faster rate of proliferation than DPSCs and differentiate into an even greater number of cells, e.g. other mesenchymal and non-mesenchymal stem cell derivatives, such as neural cells [ 130 ]. These cells possess one major disadvantage: they form a non-complete dentin/pulp-like complex in vivo. SHED do not undergo the same ethical concerns as embryonic stem cells. Both DPSCs and SHED are able to form bone-like tissues in vivo [ 131 ] and can be used for periodontal, dentin, or pulp regeneration. DPSCs and SHED can be used in treating, for example, neural deficits [ 132 ]. DPSCs alone were tested and successfully applied for alveolar bone and mandible reconstruction [ 133 ].
Periodontal ligament stem cells (PDLSCs)
These cells are used in periodontal ligament or cementum tissue regeneration. They can differentiate into mesenchymal cell lineages to produce collagen-forming cells, adipocytes, cementum tissue, Sharpey’s fibres, and osteoblast-like cells in vitro. PDLSCs exist both on the root and alveolar bone surfaces; however, on the latter, these cells have better differentiation abilities than on the former [ 134 ]. PDLSCs have become the first treatment for periodontal regeneration therapy because of their safety and efficiency [ 135 , 136 ].
Stem cells from apical papilla (SCAP)
These cells are mesenchymal structures located within immature roots. They are isolated from human immature permanent apical papilla. SCAP are the source of odontoblasts and cause apexogenesis. These stem cells can be induced in vitro to form odontoblast-like cells, neuron-like cells, or adipocytes. SCAP have a higher capacity of proliferation than DPSCs, which makes them a better choice for tissue regeneration [ 137 , 138 ].
Dental follicle stem cells (DFCs)
These cells are loose connective tissues surrounding the developing tooth germ. DFCs contain cells that can differentiate into cementoblasts, osteoblasts, and periodontal ligament cells [ 139 , 140 ]. Additionally, these cells proliferate after even more than 30 passages [ 141 ]. DFCs are most commonly extracted from the sac of a third molar. When DFCs are combined with a treated dentin matrix, they can form a root-like tissue with a pulp-dentin complex and eventually form tooth roots [ 141 ]. When DFC sheets are induced by Hertwig’s epithelial root sheath cells, they can produce periodontal tissue; thus, DFCs represent a very promising material for tooth regeneration [ 142 ].
Pulp regeneration in endodontics
Dental pulp stem cells can differentiate into odontoblasts. There are few methods that enable the regeneration of the pulp.
The first is an ex vivo method. Proper stem cells are grown on a scaffold before they are implanted into the root channel [ 143 ].
The second is an in vivo method. This method focuses on injecting stem cells into disinfected root channels after the opening of the in vivo apex. Additionally, the use of a scaffold is necessary to prevent the movement of cells towards other tissues. For now, only pulp-like structures have been created successfully.
Methods of placing stem cells into the root channel constitute are either soft scaffolding [ 144 ] or the application of stem cells in apexogenesis or apexification. Immature teeth are the best source [ 145 ]. Nerve and blood vessel network regeneration are extremely vital to keep pulp tissue healthy.
The potential of dental stem cells is mainly regarding the regeneration of damaged dentin and pulp or the repair of any perforations; in the future, it appears to be even possible to generate the whole tooth. Such an immense success would lead to the gradual replacement of implant treatments. Mandibulary and maxillary defects can be one of the most complicated dental problems for stem cells to address.
Acquiring non-dental tissue cells by dental stem cell differentiation
In 2013, it was reported that it is possible to grow teeth from stem cells obtained extra-orally, e.g. from urine [ 146 ]. Pluripotent stem cells derived from human urine were induced and generated tooth-like structures. The physical properties of the structures were similar to natural ones except for hardness [ 127 ]. Nonetheless, it appears to be a very promising technique because it is non-invasive and relatively low-cost, and somatic cells can be used instead of embryonic cells. More importantly, stem cells derived from urine did not form any tumours, and the use of autologous cells reduces the chances of rejection [ 147 ].
Use of graphene in stem cell therapy
Over recent years, graphene and its derivatives have been increasingly used as scaffold materials to mediate stem cell growth and differentiation [ 148 ]. Both graphene and graphene oxide (GO) represent high in-plane stiffness [ 149 ]. Because graphene has carbon and aromatic network, it works either covalently or non-covalently with biomolecules; in addition to its superior mechanical properties, graphene offers versatile chemistry. Graphene exhibits biocompatibility with cells and their proper adhesion. It also tested positively for enhancing the proliferation or differentiation of stem cells [ 148 ]. After positive experiments, graphene revealed great potential as a scaffold and guide for specific lineages of stem cell differentiation [ 150 ]. Graphene has been successfully used in the transplantation of hMSCs and their guided differentiation to specific cells. The acceleration skills of graphene differentiation and division were also investigated. It was discovered that graphene can serve as a platform with increased adhesion for both growth factors and differentiation chemicals. It was also discovered that π-π binding was responsible for increased adhesion and played a crucial role in inducing hMSC differentiation [ 150 ].
Therapeutic potential of extracellular vesicle-based therapies
Extracellular vesicles (EVs) can be released by virtually every cell of an organism, including stem cells [ 151 ], and are involved in intercellular communication through the delivery of their mRNAs, lipids, and proteins. As Oh et al. [ 152 ] prove, stem cells, together with their paracrine factors—exosomes—can become potential therapeutics in the treatment of, e.g. skin ageing. Exosomes are small membrane vesicles secreted by most cells (30–120 nm in diameter) [ 153 ]. When endosomes fuse with the plasma membrane, they become exosomes that have messenger RNAs (mRNAs) and microRNAs (miRNAs), some classes of non-coding RNAs (IncRNAs) and several proteins that originate from the host cell [ 154 ]. IncRNAs can bind to specific loci and create epigenetic regulators, which leads to the formation of epigenetic modifications in recipient cells. Because of this feature, exosomes are believed to be implicated in cell-to-cell communication and the progression of diseases such as cancer [ 155 ]. Recently, many studies have also shown the therapeutic use of exosomes derived from stem cells, e.g. skin damage and renal or lung injuries [ 156 ].
In skin ageing, the most important factor is exposure to UV light, called “photoageing” [ 157 ], which causes extrinsic skin damage, characterized by dryness, roughness, irregular pigmentation, lesions, and skin cancers. In intrinsic skin ageing, on the other hand, the loss of elasticity is a characteristic feature. The skin dermis consists of fibroblasts, which are responsible for the synthesis of crucial skin elements, such as procollagen or elastic fibres. These elements form either basic framework extracellular matrix constituents of the skin dermis or play a major role in tissue elasticity. Fibroblast efficiency and abundance decrease with ageing [ 158 ]. Stem cells can promote the proliferation of dermal fibroblasts by secreting cytokines such as platelet-derived growth factor (PDGF), transforming growth factor β (TGF-β), and basic fibroblast growth factor. Huh et al. [ 159 ] mentioned that a medium of human amniotic fluid-derived stem cells (hAFSC) positively affected skin regeneration after longwave UV-induced (UVA, 315–400 nm) photoageing by increasing the proliferation and migration of dermal fibroblasts. It was discovered that, in addition to the induction of fibroblast physiology, hAFSC transplantation also improved diseases in cases of renal pathology, various cancers, or stroke [ 160 , 161 ].
Oh [ 162 ] also presented another option for the treatment of skin wounds, either caused by physical damage or due to diabetic ulcers. Induced pluripotent stem cell-conditioned medium (iPSC-CM) without any animal-derived components induced dermal fibroblast proliferation and migration.
Natural cutaneous wound healing is divided into three steps: haemostasis/inflammation, proliferation, and remodelling. During the crucial step of proliferation, fibroblasts migrate and increase in number, indicating that it is a critical step in skin repair, and factors such as iPSC-CM that impact it can improve the whole cutaneous wound healing process. Paracrine actions performed by iPSCs are also important for this therapeutic effect [ 163 ]. These actions result in the secretion of cytokines such as TGF-β, interleukin (IL)-6, IL-8, monocyte chemotactic protein-1 (MCP-1), vascular endothelial growth factor (VEGF), platelet-derived growth factor-AA (PDGF-AA), and basic fibroblast growth factor (bFGF). Bae et al. [ 164 ] mentioned that TGF-β induced the migration of keratinocytes. It was also demonstrated that iPSC factors can enhance skin wound healing in vivo and in vitro when Zhou et al. [ 165 ] enhanced wound healing, even after carbon dioxide laser resurfacing in an in vivo study.
Peng et al. [ 166 ] investigated the effects of EVs derived from hESCs on in vitro cultured retinal glial, progenitor Müller cells, which are known to differentiate into retinal neurons. EVs appear heterogeneous in size and can be internalized by cultured Müller cells, and their proteins are involved in the induction and maintenance of stem cell pluripotency. These stem cell-derived vesicles were responsible for the neuronal trans-differentiation of cultured Müller cells exposed to them. However, the research article points out that the procedure was accomplished only on in vitro acquired retina.
Challenges concerning stem cell therapy
Although stem cells appear to be an ideal solution for medicine, there are still many obstacles that need to be overcome in the future. One of the first problems is ethical concern.
The most common pluripotent stem cells are ESCs. Therapies concerning their use at the beginning were, and still are, the source of ethical conflicts. The reason behind it started when, in 1998, scientists discovered the possibility of removing ESCs from human embryos. Stem cell therapy appeared to be very effective in treating many, even previously incurable, diseases. The problem was that when scientists isolated ESCs in the lab, the embryo, which had potential for becoming a human, was destroyed (Fig. 8 ). Because of this, scientists, seeing a large potential in this treatment method, focused their efforts on making it possible to isolate stem cells without endangering their source—the embryo.
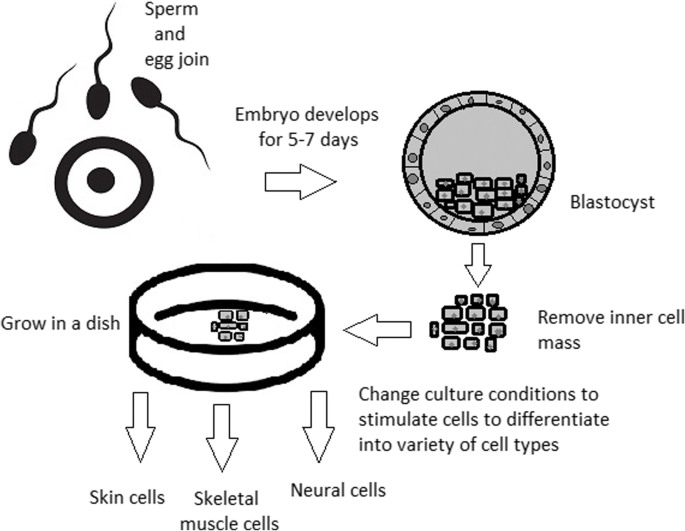
Use of inner cell mass pluripotent stem cells and their stimulation to differentiate into desired cell types
For now, while hESCs still remain an ethically debatable source of cells, they are potentially powerful tools to be used for therapeutic applications of tissue regeneration. Because of the complexity of stem cell control systems, there is still much to be learned through observations in vitro. For stem cells to become a popular and widely accessible procedure, tumour risk must be assessed. The second problem is to achieve successful immunological tolerance between stem cells and the patient’s body. For now, one of the best ideas is to use the patient’s own cells and devolve them into their pluripotent stage of development.
New cells need to have the ability to fully replace lost or malfunctioning natural cells. Additionally, there is a concern about the possibility of obtaining stem cells without the risk of morbidity or pain for either the patient or the donor. Uncontrolled proliferation and differentiation of cells after implementation must also be assessed before its use in a wide variety of regenerative procedures on living patients [ 167 ].
One of the arguments that limit the use of iPSCs is their infamous role in tumourigenicity. There is a risk that the expression of oncogenes may increase when cells are being reprogrammed. In 2008, a technique was discovered that allowed scientists to remove oncogenes after a cell achieved pluripotency, although it is not efficient yet and takes a longer amount of time. The process of reprogramming may be enhanced by deletion of the tumour suppressor gene p53, but this gene also acts as a key regulator of cancer, which makes it impossible to remove in order to avoid more mutations in the reprogrammed cell. The low efficiency of the process is another problem, which is progressively becoming reduced with each year. At first, the rate of somatic cell reprogramming in Yamanaka’s study was up to 0.1%. The use of transcription factors creates a risk of genomic insertion and further mutation of the target cell genome. For now, the only ethically acceptable operation is an injection of hESCs into mouse embryos in the case of pluripotency evaluation [ 168 ].
Stem cell obstacles in the future
Pioneering scientific and medical advances always have to be carefully policed in order to make sure they are both ethical and safe. Because stem cell therapy already has a large impact on many aspects of life, it should not be treated differently.
Currently, there are several challenges concerning stem cells. First, the most important one is about fully understanding the mechanism by which stem cells function first in animal models. This step cannot be avoided. For the widespread, global acceptance of the procedure, fear of the unknown is the greatest challenge to overcome.
The efficiency of stem cell-directed differentiation must be improved to make stem cells more reliable and trustworthy for a regular patient. The scale of the procedure is another challenge. Future stem cell therapies may be a significant obstacle. Transplanting new, fully functional organs made by stem cell therapy would require the creation of millions of working and biologically accurate cooperating cells. Bringing such complicated procedures into general, widespread regenerative medicine will require interdisciplinary and international collaboration.
The identification and proper isolation of stem cells from a patient’s tissues is another challenge. Immunological rejection is a major barrier to successful stem cell transplantation. With certain types of stem cells and procedures, the immune system may recognize transplanted cells as foreign bodies, triggering an immune reaction resulting in transplant or cell rejection.
One of the ideas that can make stem cells a “failsafe” is about implementing a self-destruct option if they become dangerous. Further development and versatility of stem cells may cause reduction of treatment costs for people suffering from currently incurable diseases. When facing certain organ failure, instead of undergoing extraordinarily expensive drug treatment, the patient would be able to utilize stem cell therapy. The effect of a successful operation would be immediate, and the patient would avoid chronic pharmacological treatment and its inevitable side effects.
Although these challenges facing stem cell science can be overwhelming, the field is making great advances each day. Stem cell therapy is already available for treating several diseases and conditions. Their impact on future medicine appears to be significant.
After several decades of experiments, stem cell therapy is becoming a magnificent game changer for medicine. With each experiment, the capabilities of stem cells are growing, although there are still many obstacles to overcome. Regardless, the influence of stem cells in regenerative medicine and transplantology is immense. Currently, untreatable neurodegenerative diseases have the possibility of becoming treatable with stem cell therapy. Induced pluripotency enables the use of a patient’s own cells. Tissue banks are becoming increasingly popular, as they gather cells that are the source of regenerative medicine in a struggle against present and future diseases. With stem cell therapy and all its regenerative benefits, we are better able to prolong human life than at any time in history.
Abbreviations
Basic fibroblast growth factor
Bone morphogenic proteins
Dental follicle stem cells
Dental pulp stem cells
Embryonic bodies
Embryonic stem cells
Fibroblast growth factors
Good Manufacturing Practice
Graphene oxide
Human amniotic fluid-derived stem cells
Human embryonic stem cells
Human foreskin fibroblasts
Inner cell mass
Non-coding RNA
Induced pluripotent stem cells
In vitro fertilization
Knockout serum replacement
Leukaemia inhibitory factor
Monocyte chemotactic protein-1
Fibroblasts
Messenger RNA
Mesenchymal stem cells of dental pulp
Myogenic differentiation
Osteoarthritis
Octamer-binding transcription factor 3 and 4
Platelet-derived growth factor
Platelet-derived growth factor-AA
Periodontal ligament stem cells
Rho-associated protein kinase
Stem cells from apical papilla
Stem cells of human exfoliated deciduous teeth
Synthetic Serum Substitute
Trophectoderm
Vascular endothelial growth factor
Transforming growth factors
Sukoyan MA, Vatolin SY, et al. Embryonic stem cells derived from morulae, inner cell mass, and blastocysts of mink: comparisons of their pluripotencies. Embryo Dev. 1993;36(2):148–58
Larijani B, Esfahani EN, Amini P, Nikbin B, Alimoghaddam K, Amiri S, Malekzadeh R, Yazdi NM, Ghodsi M, Dowlati Y, Sahraian MA, Ghavamzadeh A. Stem cell therapy in treatment of different diseases. Acta Medica Iranica. 2012:79–96 https://www.ncbi.nlm.nih.gov/pubmed/22359076 .
Sullivan S, Stacey GN, Akazawa C, et al. Quality guidelines for clinical-grade human induced pluripotent stem cell lines. Regenerative Med. 2018; https://doi.org/10.2217/rme-2018-0095 .
Amps K, Andrews PW, et al. Screening ethnically diverse human embryonic stem cells identifies a chromosome 20 minimal amplicon conferring growth advantage. Nat. Biotechnol. 2011; 29 (12):1121–44.
Google Scholar
Amit M, Itskovitz-Eldor J. Atlas of human pluripotent stem cells: derivation and culturing. New York: Humana Press; 2012.
Ludwig TE, Bergendahl V, Levenstein ME, Yu J, Probasco MD, Thomson JA. Feeder-independent culture of human embryonic stem cells. Nat Methods. 2006;3:637–46.
CAS PubMed Google Scholar
Kang MI. Transitional CpG methylation between promoters and retroelements of tissue-specific genes during human mesenchymal cell differentiation. J. Cell Biochem. 2007;102:224–39.
Vaes B, Craeye D, Pinxteren J. Quality control during manufacture of a stem cell therapeutic. BioProcess Int. 2012;10:50–5.
Bloushtain-Qimron N. Epigenetic patterns of embryonic and adult stem cells. Cell Cycle. 2009;8:809–17.
Brindley DA. Peak serum: implications of serum supply for cell therapy manufacturing. Regenerative Medicine. 2012;7:809–17.
Solter D, Knowles BB. Immunosurgery of mouse blastocyst. Proc Natl Acad Sci U S A. 1975;72:5099–102.
CAS PubMed PubMed Central Google Scholar
Hoepfl G, Gassmann M, Desbaillets I. Differentiating embryonic stem cells into embryoid bodies. Methods Mole Biol. 2004;254:79–98 https://doi.org/10.1385/1-59259-741-6:079 .
Lim WF, Inoue-Yokoo T, Tan KS, Lai MI, Sugiyama D. Hematopoietic cell differentiation from embryonic and induced pluripotent stem cells. Stem Cell Res Ther. 2013;4(3):71. https://doi.org/10.1186/scrt222 .
Article CAS PubMed PubMed Central Google Scholar
Mohr JC, de Pablo JJ, Palecek SP. 3-D microwell culture of human embryonic stem cells. Biomaterials. 2006;27(36):6032–42. https://doi.org/10.1016/j.biomaterials.2006.07.012 .
Article CAS PubMed Google Scholar
Doetschman TC, Eistetter H, Katz M, Schmidt W, Kemler R. The in vitro development of blastocyst-derived embryonic stem cell lines: formation of the visceral yolk sac, blood islands, and myocardium. J Embryol Exp Morphol. 1985;87:27–45.
Kurosawa HY. Methods for inducing embryoid body formation: in vitro differentiation system of embryonic stem cells. J Biosci Bioeng. 2007;103:389–98.
Heins N, Englund MC, Sjoblom C, Dahl U, Tonning A, Bergh C, Lindahl A, Hanson C, Semb H. Derivation, characterization, and differentiation of human embryonic stem cells. Stem Cells. 2004;22:367–76.
Rosowski KA, Mertz AF, Norcross S, Dufresne ER, Horsley V. Edges of human embryonic stem cell colonies display distinct mechanical properties and differentiation potential. Sci Rep. 2015;5:Article number:14218.
PubMed Google Scholar
Chung Y, Klimanskaya I, Becker S, Li T, Maserati M, Lu SJ, Zdravkovic T, Ilic D, Genbacev O, Fisher S, Krtolica A, Lanza R. Human embryonic stem cell lines generated without embryo destruction. Cell Stem Cell. 2008;2:113–7.
Zhang X, Stojkovic P, Przyborski S, Cooke M, Armstrong L, Lako M, Stojkovic M. Derivation of human embryonic stem cells from developing and arrested embryos. Stem Cells. 2006;24:2669–76.
Beers J, Gulbranson DR, George N, Siniscalchi LI, Jones J, Thomson JA, Chen G. Passaging and colony expansion of human pluripotent stem cells by enzyme-free dissociation in chemically defined culture conditions. Nat Protoc. 2012;7:2029–40.
Ellerström C, Hyllner J, Strehl R. single cell enzymatic dissociation of human embryonic stem cells: a straightforward, robust, and standardized culture method. In: Turksen K, editor. Human embryonic stem cell protocols. Methods in molecular biology: Humana Press; 2009. p. 584.
Heng BC, Liu H, Ge Z, Cao T. Mechanical dissociation of human embryonic stem cell colonies by manual scraping after collagenase treatment is much more detrimental to cellular viability than is trypsinization with gentle pipetting. Biotechnol Appl Biochem. 2010;47(1):33–7.
Ellerstrom C, Strehl R, Noaksson K, Hyllner J, Semb H. Facilitated expansion of human embryonic stem cells by single-cell enzymatic dissociation. Stem Cells. 2007;25:1690–6.
Brimble SN, Zeng X, Weiler DA, Luo Y, Liu Y, Lyons IG, Freed WJ, Robins AJ, Rao MS, Schulz TC. Karyotypic stability, genotyping, differentiation, feeder-free maintenance, and gene expression sampling in three human embryonic stem cell lines deri. Stem Cells Dev. 2004;13:585–97.
Watanabe K, Ueno M, Kamiya D, Nishiyama A, Matsumura M, Wataya T, Takahashi JB, Nishikawa S, Nishikawa S, Muguruma K, Sasai Y. A ROCK inhibitor permits survival of dissociated human embryonic stem cells. Nat Biotechnol. 2007;25:681–6.
Nie Y, Walsh P, Clarke DL, Rowley JA, Fellner T. Scalable passaging of adherent human pluripotent stem cells. 2014. https://doi.org/10.1371/journal.pone.0088012 .
Thomson JA, Itskovitz-Eldor J, Shapiro SS, Waknitz MA, Swiergiel JJ, Marshall VS, Jones JM. Embryonic stem cell lines derived from human blastocysts. Science. 1998;282:1145–7.
Martin MJ, Muotri A, Gage F, Varki A. Human embryonic stem cellsexpress an immunogenic nonhuman sialic acid. Nat. Med. 2005;11:228–32.
Smith AG, Heath JK, Donaldson DD, Wong GG, Moreau J, Stahl M, Rogers D. Inhibition of pluripotential embryonic stem cell differentiation by purified polypeptides. Nature. 1988;336(6200):688–90. https://doi.org/10.1038/336688a0 .
Xu C, Inokuma MS, Denham J, Golds K, Kundu P, Gold JD, Carpenter MK. Feeder-free growth of undifferentiated human embryonic stem cells. Nature Biotechnol. 2001;19:971–4. https://doi.org/10.1038/nbt1001-971 .
Article CAS Google Scholar
Weathersbee PS, Pool TB, Ord T. Synthetic serum substitute (SSS): a globulin-enriched protein supplement for human embryo culture. J. Assist Reprod Genet. 1995;12:354–60.
Chen G, Gulbranson DR, Hou Z, Bolin JM, Ruotti V, Probasco MD, Smuga-Otto K, Howden SE, Diol NR, Propson NE, Wagner R, Lee GO, Antosiewicz-Bourget J, Teng JM, Thomson JA. Chemically defined conditions for human iPSC derivation and culture. Nat. Methods. 2011;8:424–9.
Sommer CA, Mostoslavsky G. Experimental approaches for the generation of induced pluripotent stem cells. Stem Cell Res Ther. 2010;1:26.
PubMed PubMed Central Google Scholar
Takahashi K, Yamanaka S. Induced pluripotent stem cells in medicine and biology. Development. 2013;140(12):2457–61 https://doi.org/10.1242/dev.092551 .
Shi D, Lu F, Wei Y, et al. Buffalos ( Bubalus bubalis ) cloned by nuclear transfer of somatic cells. Biol. Reprod. 2007;77:285–91. https://doi.org/10.1095/biolreprod.107.060210 .
Gurdon JB. The developmental capacity of nuclei taken from intestinal epithelium cells of feeding tadpoles. Development. 1962;10:622–40 http://dev.biologists.org/content/10/4/622 .
CAS Google Scholar
Kain K. The birth of cloning: an interview with John Gurdon. Dis Model Mech. 2009;2(1–2):9–10. https://doi.org/10.1242/dmm.002014 .
Article PubMed Central Google Scholar
Davis RL, Weintraub H, Lassar AB. Expression of a single transfected cDNA converts fibroblasts to myoblasts. Cell. 1987;24(51(6)):987–1000.
Quinlan AR, Boland MJ, Leibowitz ML, et al. Genome sequencing of mouse induced pluripotent stem cells reveals retroelement stability and infrequent DNA rearrangement during reprogramming. Cell Stem Cell. 2011;9(4):366–73.
Maherali N, Sridharan R, Xie W, Utika LJ, Eminli S, Arnold K, Stadtfeld M, Yachechko R, Tchieu J, Jaenisch R, Plath K, Hochedlinger K. Directly reprogrammed fibroblasts show global epigenetic remodeling and widespread tissue contribution. Cell Stem Cell. 2007;1:55–70.
Ohi Y, Qin H, Hong C, Blouin L, Polo JM, Guo T, Qi Z, Downey SL, Manos PD, Rossi DJ, Yu J, Hebrok M, Hochedlinger K, Costello JF, Song JS, Ramalho-Santos M. Incomplete DNA methylation underlines a transcriptional memory of somatic cells in human IPS cells. Nat Cell Biol. 2011;13:541–9.
Zhou Q, Brown J, Kanarek A, Rajagopal J, Melton DA. In vivo reprogramming of adult pancreatic exocrine cells to beta-cells. Nature. 2008;455:627–32 https://doi.org/10.1038/nature07314 .
Hilfiker A, Kasper C, Hass R, Haverich A. Mesenchymal stem cells and progenitor cells in connective tissue engineering and regenerative medicine: is there a future for transplantation? Langenbecks Arch Surg. 2011;396:489–97.
Zhang Wendy, Y., de Almeida Patricia, E., and Wu Joseph, C. Teratoma formation: a tool for monitoring pluripotency in stem cell research. StemBook, ed. The Stem Cell Research Community . June 12, 2012. https://doi.org/10.3824/stembook.1.53.1 .
Narsinh KH, Sun N, Sanchez-Freire V, et al. Single cell transcriptional profiling reveals heterogeneity of human induced pluripotent stem cells. J Clin Invest. 2011;121(3):1217–21.
Gertow K, Przyborski S, Loring JF, Auerbach JM, Epifano O, Otonkoski T, Damjanov I, AhrlundRichter L. Isolation of human embryonic stem cell-derived teratomas for the assessment of pluripotency. Curr Protoc Stem Cell Biol . 2007, Chapter 1, Unit 1B 4. 3: 1B.4.1-1B.4.29.
Cooke MJ, Stojkovic M, Przyborski SA. Growth of teratomas derived from human pluripotent stem cells is influenced by the graft site. Stem Cells Dev. 2006;15(2):254–9.
Przyborski SA. Differentiation of human embryonic stem cells after transplantation in immune-deficient mice. Stem Cells. 2005;23:1242–50.
Tannenbaum SE, Turetsky TT, Singer O, Aizenman E, Kirshberg S, Ilouz N, Gil Y, Berman-Zaken Y, Perlman TS, Geva N, Levy O, Arbell D, Simon A, Ben-Meir A, Shufaro Y, Laufer N, Reubinoff BE. Derivation of xeno-free and GMP-grade human embryonic stem cells- platforms for future clinical applications. PLoS One. 2012;7:e35325.
Cohen DE, Melton D. Turning straw into gold: directing cell fate for regenerative medicine. Nat Rev Genet. 2011;12:243–52.
Hwang NS, Varghese S, Elisseeff J. Controlled differentiation of stem cells. Adv Drug Deliv Rev. 2007;60(2):199–214. https://doi.org/10.1016/j.addr.2007.08.036 .
Turner N, Grose R. Fibroblast growth factor signalling: from development to cancer. Nat Rev Cancer. 2010;10:116–29.
Rao TP, Kuhl M. An updated overview on Wnt signaling pathways: a prelude for more. Circ Res. 2010;106:1798–806.
Moustakas A, Heldin CH. The regulation of TGFbeta signal transduction. Development. 2009;136:3699–714.
Efthymiou AG, Chen G, Rao M, Chen G, Boehm M. Self-renewal and cell lineage differentiation strategies in human embryonic stem cells and induced pluripotent stem cells. Expert Opin Biol Ther. 2014;14:1333–44.
Yang L, Soonpaa MH, Adler ED, Roepke TK, Kattman SJ, Kennedy M, Henckaerts E, Bonham K, Abbott GW, Linden RM, Field LJ, Keller GM. Human cardiovascular progenitor cells develop from a KDRþembryonic-stem-cell-derived population. Nature. 2008;453:524–8.
Kroon E, Martinson LA, Kadoya K, Bang AG, Kelly OG, Eliazer S, Young H, Richardson M, Smart NG, Cunningham J, Agulnick AD, D’amour KA, Carpenter MK, Baetge EE. Pancreatic endoderm derived from human embryonic stem cells generates glucose-responsive insulin-secreting cells in vivo. Nat Biotechnol. 2008;26(4):443–52. https://doi.org/10.1038/nbt1393 .
Vallier L, Reynolds D, Pedersen RA. Nodal inhibits differentiation of human embryonic stem cells along the neuroectodermal default pathway. Dev Biol. 2004;275:403–21.
Burridge PW, Zambidis ET. Highly efficient directed differentiation of human induced pluripotent stem cells into cardiomyocytes. Methods Mol Biol. 2013;997:149–61.
Cai J, Zhao Y, Liu Y, Ye F, Song Z, Qin H, Meng S, Chen Y, Zhou R, Song X, Guo Y, Ding M, Deng H. Directed differentiation of human embryonic stem cells into functional hepatic cells. Hepatology. 2007;45:1229–39.
Takasato M, Er PX, Becroft M, Vanslambrouck JM, Stanley EG, Elefanty AG, Little MH. Directing human embryonic stem cell differentiation towards a renal lineage generates a selforganizing kidney. Nat Cell Biol. 2014;16:118–26.
Huang SX, Islam MN, O’Neill J, Hu Z, Yang YG, Chen YW, Mumau M, Green MD, VunjakNovakovic G, Bhattacharya J, Snoeck HW. Efficient generation of lung and airway epithelial cells from human pluripotent stem cells. Nat Biotechnol. 2014;32:84–91.
Kadzik RS, Morrisey EE. Directing lung endoderm differentiation in pluripotent stem cells. Cell Stem Cell. 2012;10:355–61.
Wichterle H, Lieberam I, Porter JA, Jessell TM. Directed differentiation of embryonic stem cells into motor neurons. Cell. 2002;110:385–97.
Spence JR, Mayhew CN, Rankin SA, Kuhar MF, Vallance JE, Tolle K, Hoskins EE, Kalinichenko VV, Wells SI, Zorn AM, Shroyer NF, Wells JM. Directed differentiation of human pluripotent stem cells into intestinal tissue in vitro. Nature. 2011;470:105–9.
Oldershaw RA, Baxter MA, Lowe ET, Bates N, Grady LM, Soncin F, Brison DR, Hardingham TE, Kimber SJ. Directed differentiation of human embryonic stem cells toward chondrocytes. Nat Biotechnol. 2010;28:1187–94.
Jun Cai, Ann DeLaForest, Joseph Fisher, Amanda Urick, Thomas Wagner, Kirk Twaroski, Max Cayo, Masato Nagaoka, Stephen A Duncan. Protocol for directed differentiation of human pluripotent stem cells toward a hepatocyte fate. 2012. DOI: https://doi.org/10.3824/stembook.1.52.1 .
Frank-Kamenetsky M, Zhang XM, Bottega S, Guicherit O, Wichterle H, Dudek H, Bumcrot D, Wang FY, Jones S, Shulok J, Rubin LL, Porter JA. Small-molecule modulators of hedgehog signaling: identification and characterization of smoothened agonists and antagonists. J Biol. 2002;1:10.
Oshima K, Shin K, Diensthuber M, Peng AW, Ricci AJ, Heller S. Mechanosensitive hair celllike cells from embryonic and induced pluripotent stem cells. Cell. 2010;141:704–16.
Osakada F, Jin ZB, Hirami Y, Ikeda H, Danjyo T, Watanabe K, Sasai Y, Takahashi M. In vitro differentiation of retinal cells from human pluripotent stem cells by small-molecule induction. J Cell Sci. 2009;122:3169–79.
Kshitiz PJ, Kim P, Helen W, Engler AJ, Levchenko A, Kim DH. Control of stem cell fate and function by engineering physical microenvironments. Intergr Biol (Camb). 2012;4:1008–18.
Amps K, Andrews PW, Anyfantis G, Armstrong L, Avery S, Baharvand H, Baker J, Baker D, Munoz MB, Beil S, Benvenisty N, Ben-Yosef D, Biancotti JC, Bosman A, Brena RM, Brison D, Caisander G, Camarasa MV, Chen J, ChiaoE CYM, Choo AB, Collins D, et al. Screening ethnically diverse human embryonic stem cells identifies a chromosome 20 minimal amplicon conferring growth advantage. Nat Biotechnol. 2011;29:1132–44.
Nukaya D, Minami K, Hoshikawa R, Yokoi N, Seino S. Preferential gene expression and epigenetic memory of induced pluripotent stem cells derived from mouse pancreas. Genes Cells. 2015;20:367–81.
Murdoch A, Braude P, Courtney A, Brison D, Hunt C, Lawford-Davies J, Moore H, Stacey G, Sethe S, Procurement Working Group Of National Clinical H, E. S. C. F, National Clinical H, E. S. C. F. The procurement of cells for the derivation of human embryonic stem cell lines for therapeutic use: recommendations for good practice. Stem Cell Rev. 2012;8:91–9.
Hewitson H, Wood V, Kadeva N, Cornwell G, Codognotto S, Stephenson E, Ilic D. Generation of KCL035 research grade human embryonic stem cell line carrying a mutation in HBB gene. Stem Cell Res. 2016;16:210–2.
Daley GQ, Hyun I, Apperley JF, Barker RA, Benvenisty N, Bredenoord AL, Breuer CK, Caulfield T, Cedars MI, Frey-Vasconcells J, Heslop HE, Jin Y, Lee RT, Mccabe C, Munsie M, Murry CE, Piantadosi S, Rao M, Rooke HM, Sipp D, Studer L, Sugarman J, et al. Setting global standards for stem cell research and clinical translation: the 2016 ISSCR guidelines. Stem Cell Rep. 2016;6:787–97.
Takahashi K, Yamanaka S. Induction of pluripotent stem cells from mouse embryonic and adult fibroblast cultures by defined factors. Cell. 2006;126(4):663–76. https://doi.org/10.1016/j.cell.2006.07.024 .
Loh YH, Wu Q, Chew JL, Vega VB, Zhang W, Chen X, Bourque G, George J, Leong B, Liu J, et al. The Oct4 and Nanog transcription network regulates pluripotency in mouse embryonic stem cells. Nat Genet. 2006;38:431–40.
Menasche P, Vanneaux V, Hagege A, Bel A, Cholley B, Cacciapuoti I, Parouchev A, Benhamouda N, Tachdjian G, Tosca L, Trouvin JH, Fabreguettes JR, Bellamy V, Guillemain R, SuberbielleBoissel C, Tartour E, Desnos M, Larghero J. Human embryonic stem cell-derived cardiac progenitors for severe heart failure treatment: first clinical case report. Eur Heart J. 2015;36:2011–7.
Schwartz SD, Regillo CD, Lam BL, Eliott D, Rosenfeld PJ, Gregori NZ, Hubschman JP, Davis JL, Heilwell G, Spirn M, Maguire J, Gay R, Bateman J, Ostrick RM, Morris D, Vincent M, Anglade E, Del Priore LV, Lanza R. Human embryonic stem cell-derived retinal pigment epithelium in patients with age-related macular degeneration and Stargardt’s macular dystrophy: follow-up of two open-label phase 1/2 studies. Lancet. 2015;385:509–16.
Ilic D, Ogilvie C. Concise review: human embryonic stem cells-what have we done? What are we doing? Where are we going? Stem Cells. 2017;35:17–25.
Rocha V, et al. Clinical use of umbilical cord blood hematopoietic stem cells. Biol Blood Marrow Transplant. 2006;12(1):34–4.
Longo UG, Ronga M, Maffulli N. Sports Med Arthrosc 17:112–126. Achilles tendinopathy. Sports Med Arthrosc. 2009;17:112–26.
Tempfer H, Lehner C, Grütz M, Gehwolf R, Traweger A. Biological augmentation for tendon repair: lessons to be learned from development, disease, and tendon stem cell research. In: Gimble J, Marolt D, Oreffo R, Redl H, Wolbank S, editors. Cell engineering and regeneration. Reference Series in Biomedical Engineering. Cham: Springer; 2017.
Goldring MB, Goldring SR. Osteoarthritis. J Cell Physiol. 2007;213:626–34.
Widuchowski W, Widuchowski J, Trzaska T. Articular cartilage defects: study of 25,124 knee arthroscopies. Knee. 2007;14:177–82.
Li R, Lin Q-X, Liang X-Z, Liu G-B, et al. Stem cell therapy for treating osteonecrosis of the femoral head: from clinical applications to related basic research. Stem Cell Res Therapy. 2018;9:291 https://doi.org/10.1186/s13287-018-1018-7 .
Gangji V, De Maertelaer V, Hauzeur JP. Autologous bone marrow cell implantation in the treatment of non-traumatic osteonecrosis of the femoral head: five year follow-up of a prospective controlled study. Bone. 2011;49(5):1005–9.
Zhao D, Cui D, Wang B, Tian F, Guo L, Yang L, et al. Treatment of early stage osteonecrosis of the femoral head with autologous implantation of bone marrow-derived and cultured mesenchymal stem cells. Bone. 2012;50(1):325–30.
Sen RK, Tripathy SK, Aggarwal S, Marwaha N, Sharma RR, Khandelwal N. Early results of core decompression and autologous bone marrow mononuclear cells instillation in femoral head osteonecrosis: a randomized control study. J Arthroplast. 2012;27(5):679–86.
Lapasset L, Milhavet O, Prieur A, Besnard E, Babled A, Aït-Hamou N, Leschik J, Pellestor F, Ramirez JM, De Vos J, Lehmann S, Lemaitre JM. Rejuvenating senescent and centenarian human cells by reprogramming through the pluripotent state. Genes Dev. 2011;25:2248–53.
Sahin E, Depinho RA. Linking functional decline of telomeres, mitochondria and stem cells during ageing. Nature. 2010;464:520–8.
Petkovich DA, Podolskiy DI, Lobanov AV, Lee SG, Miller RA, Gladyshev VN. Using DNA methylation profiling to evaluate biological age and longevity interventions. Cell Metab. 2017;25:954–60 https://doi.org/10.1016/j.cmet.2017.03.016 .
Gerontology, Rejuvenation by cell reprogramming: a new horizon in. Rodolfo G. Goya, Marianne Lehmann, Priscila Chiavellini, Martina Canatelli-Mallat, Claudia B. Hereñú and Oscar A. Brown. Stem Cell Res Therapy . 2018, 9:349. https://doi.org/10.1186/s13287-018-1075-y .
Ocampo A, Reddy P, Martinez-Redondo P, Platero-Luengo A, Hatanaka F, Hishida T, Li M, Lam D, Kurita M, Beyret E, Araoka T, Vazquez-Ferrer E, Donoso D, Roman JLXJ, Rodriguez-Esteban C, Nuñez G, Nuñez Delicado E, Campistol JM, Guillen I, Guillen P, Izpisua. In vivo amelioration of age-associated hallmarks by partial reprogramming. Cell. 2016;167:1719–33.
de Lázaro I, Cossu G, Kostarelos K. Transient transcription factor (OSKM) expression is key towards clinical translation of in vivo cell reprogramming. EMBO Mol Med. 2017;9:733–6.
Sun S, Li ZQ, Glencer P, Cai BC, Zhang XM, Yang J, Li XR. Bringing the age-related macular degeneration high-risk allele age-related maculopathy susceptibility 2 into focus with stem cell technology. Stem Cell Res Ther. 2017;8:135 https://doi.org/10.1186/s13287-017-0584-4 .
Liu J. Induced pluripotent stem cell-derived neural stem cells: new hope for stroke? Stem Cell Res Ther. 2013;4:115 https://doi.org/10.1186/scrt326 .
Shahjalal HM, Dayem AA, Lim KM, Jeon TI, Cho SG. Generation of pancreatic β cells for treatment of diabetes: advances and challenges. Stem Cell ResTher. 2018;9:355 https://doi.org/10.1186/s13287-018-1099-3 .
Kroon E, Martinson LA, et al. Pancreatic endoderm derived from human embryonic stem cells generates glucose-responsive insulin-secreting cells in vivo. Nat Biotechnol. 2008;26;443–52.
Arora V, Pooja A, Munshi AK. Banking stem cells from human exfoliated deciduous teeth. J Clin Pediatr Dent. 2009;33(4):289–94.
Mao JJ. Stem cells and the future of dental care. New York State Dental J. 2008;74(2):21–4.
Reznick, Jay B. Continuing education: stem cells: emerging medical and dental therapies for the dental Professional. Dentaltown Magazine . 2008, pp. 42–53.
Arthur A, Rychkov G, Shi S, Koblar SA, Gronthos S. Adult human dental pulp stem cells differentiate toward functionally active neurons under appropriate environmental cues. Stem Cells. 2008;26(7):1787–95.
Cordeiro MM, Dong Z, Kaneko T, Zhang Z, Miyazawa M, Shi S, Smith A. Dental pulp tissue engineering with stem cells from exfoliated. J Endod. 2008;34(8):962–9.
Hayashi K, Ohta H, Kurimoto K, Aramaki S, Saitou M. Reconstitution of the mouse germ cell specification pathway in culture by pluripotent stem cells. Cell. 2011;146(4):519–32. https://doi.org/10.1016/j.cell.2011.06.052 .
Sadri-Ardekani H, Atala A. Testicular tissue cryopreservation and spermatogonial stem cell transplantation to restore fertility: from bench to bedside. Stem Cell ResTher. 2014;5:68 https://doi.org/10.1186/scrt457 .
Zhang Q, Xu M, Yao X, Li T, Wang Q, Lai D. Human amniotic epithelial cells inhibit granulosa cell apoptosis induced by chemotherapy and restore the fertility. Stem Cell Res Ther. 2015;6:152 https://doi.org/10.1186/s13287-015-0148-4 .
Ma DK, Bonaguidi MA, Ming GL, Song H. Adult neural stem cells in the mammalian central nervous system. Cell Res. 2009;19:672–82. https://doi.org/10.1038/cr.2009.56 .
Dantuma E, Merchant S, Sugaya K. Stem cells for the treatment of neurodegenerative diseases. Stem Cell ResTher. 2010;1:37 https://doi.org/10.1186/scrt37 .
Wang Q, Matsumoto Y, Shindo T, Miyake K, Shindo A, Kawanishi M, Kawai N, Tamiya T, Nagao S. Neural stem cells transplantation in cortex in a mouse model of Alzheimer’s disease. J Med Invest. 2006;53:61–9. https://doi.org/10.2152/jmi.53.61 .
Article PubMed Google Scholar
Moghadam FH, Alaie H, Karbalaie K, Tanhaei S, Nasr Esfahani MH, Baharvand H. Transplantation of primed or unprimed mouse embryonic stem cell-derived neural precursor cells improves cognitive function in Alzheimerian rats. Differentiation. 2009;78:59–68. https://doi.org/10.1016/j.diff.2009.06.005 .
Byrne JA. Developing neural stem cell-based treatments for neurodegenerative diseases. Stem Cell ResTher. 2014;5:72. https://doi.org/10.1186/scrt461 .
Awe JP, Lee PC, Ramathal C, Vega-Crespo A, Durruthy-Durruthy J, Cooper A, Karumbayaram S, Lowry WE, Clark AT, Zack JA, Sebastiano V, Kohn DB, Pyle AD, Martin MG, Lipshutz GS, Phelps PE, Pera RA, Byrne JA. Generation and characterization of transgene-free human induced pluripotent stem cells and conversion to putative clinical-grade status. Stem Cell Res Ther. 2013;4:87. https://doi.org/10.1186/scrt246 .
Peng J, Zeng X. The role of induced pluripotent stem cells in regenerative medicine: neurodegenerative diseases. Stem Cell ResTher. 2011;2:32. https://doi.org/10.1186/scrt73 .
Wright BL, Barker RA. Established and emerging therapies for Huntington’s disease. 2007;7(6):579–87 https://www.ncbi.nlm.nih.gov/pubmed/17896994/579-87 .
Lin NH, Gronthos S, Bartold PM. Stem cells and periodontal regeneration. Aust Dent J. 2008;53:108–21.
Seo BM, Miura M, Gronthos S, Bartold PM, Batouli S, Brahim J, et al. Investigation of multipotent postnatal stem cells from human periodontal ligament. Lancet. 2004;364:149–55.
Ramseier CA, Abramson ZR, Jin Q, Giannobile WV. Gene therapeutics for periodontal regenerative medicine. Dent Clin North Am. 2006;50:245–63.
Shi S, Bartold PM, Miura M, Seo BM, Robey PG, Gronthos S. The efficacy of mesenchymal stem cells to regenerate and repair dental structures. OrthodCraniofac Res. 2005;8:191–9.
Iohara K, Nakashima M, Ito M, Ishikawa M, Nakasima A, Akamine A. Dentin regeneration by dental pulp stem cell therapy with recombinant human bone morphogenetic protein. J Dent Res. 2004;83:590–5.
Hu B, Unda F, Bopp-Kuchler S, Jimenez L, Wang XJ, Haikel Y, et al. Bone marrow cells can give rise to ameloblast-like cells. J Dent Res. 2006;85:416–21.
Liu Y, Liu W, Hu C, Xue Z, Wang G, Ding B, Luo H, Tang L, Kong X, Chen X, Liu N, Ding Y, Jin Y. MiR-17 modulates osteogenic differentiation through a coherent feed-forward loop in mesenchymal stem cells isolated from periodontal ligaments of patients with periodontitis. Stem Cells. 2011;29(11):1804–16. https://doi.org/10.1002/stem.728 .
Raspini G, Wolff J, Helminen M, Raspini G, Raspini M, Sándor GK. Dental stem cells harvested from third molars combined with bioactive glass can induce signs of bone formation in vitro. J Oral Maxillofac Res. 2018;9(1):e2. Published 2018 Mar 31. https://doi.org/10.5037/jomr.2018.9102 .
Christodoulou I, Goulielmaki M, Devetzi M, Panagiotidis M, Koliakos G, Zoumpourlis V. Mesenchymal stem cells in preclinical cancer cytotherapy: a systematic review. Stem Cell Res Ther. 2018;9(1;336). https://doi.org/10.1186/s13287-018-1078-8 .
Bansal R, Jain A. Current overview on dental stem cells applications in regenerative dentistry. J Nat Sci Biol Med. 2015;6(1):29–34. https://doi.org/10.4103/0976-9668.149074 .
Article PubMed PubMed Central Google Scholar
Edgar Ledesma-Martínez, Víctor Manuel Mendoza-Núñez, Edelmiro Santiago-Osorio. Mesenchymal stem cells derived from dental pulp: a review. Stem Cells Int . 2016, 4,709,572, p. doi: https://doi.org/10.1155/2016/4709572 ].
Grzech-Leśniak K. Making use of lasers in periodontal treatment: a new gold standard? Photomed Laser Surg. 2017;35(10):513–4.
Miura M, Gronthos S, Zhao M, Lu B, Fisher LW, Robey PG, Shi S. SHED: stem cells from human exfoliated deciduous teeth. Proc Natl Acad Sci U S A. 2003;100(10):5807–12. https://doi.org/10.1073/pnas.0937635100 .
Yasui T, Mabuchi Y, Toriumi H, Ebine T, Niibe K, Houlihan DD, Morikawa S, Onizawa K, Kawana H, Akazawa C, Suzuki N, Nakagawa T, Okano H, Matsuzaki Y. Purified human dental pulp stem cells promote osteogenic regeneration. J Dent Res. 2016;95(2):206–14. https://doi.org/10.1177/0022034515610748 .
Yamamoto A, Sakai K, Matsubara K, Kano F, Ueda M. Multifaceted neuro-regenerative activities of human dental pulp stem cells for functional recovery after spinal cord injury. Neurosci Res. 2014;78:16–20. https://doi.org/10.1016/j.neures.2013.10.010 .
d’Aquino R, De Rosa A, Lanza V, Tirino V, Laino L, Graziano A, Desiderio V, Laino G, Papaccio G. Human mandible bone defect repair by the grafting of dental pulp stem/progenitor cells and collagen sponge biocomplexes. Eur Cell Mater. 2009;12, PMID: 19908196:75–83.
Wang L, Shen H, Zheng W, Tang L, Yang Z, Gao Y, Yang Q, Wang C, Duan Y, Jin Y. Characterization of stem cells from alveolar periodontal ligament. Tissue Eng. Part A. 2011;17(7–8):1015–26. https://doi.org/10.1089/ten.tea.2010.0140 .
Iwata T, Yamato M, Zhang Z, Mukobata S, Washio K, Ando T, Feijen J, Okano T, Ishikawa I. Validation of human periodontal ligament-derived cells as a reliable source for cytotherapeutic use. J Clin Periodontol. 2010;37(12):1088–99. https://doi.org/10.1111/j.1600-051X.2010.01597.x .
Chen F-M, Gao L-N, Tian B-M, Zhang X-Y, Zhang Y-J, Dong G-Y, Lu H, et al. Treatment of periodontal intrabony defects using autologous periodontal ligament stem cells: a randomized clinical trial. Stem Cell Res Ther. 2016;7:33. https://doi.org/10.1186/s13287-016-0288-1 .
Bakopoulou A, Leyhausen G, Volk J, Tsiftsoglou A, Garefis P, Koidis P, Geurtsen W. Comparative analysis of in vitro osteo/odontogenic differentiation potential of human dental pulp stem cells (DPSCs) and stem cells from the apical papilla (SCAP). Arch Oral Biol. 2011;56(7):709–21. https://doi.org/10.1016/j.archoralbio.2010.12.008 .
Han C, Yang Z, Zhou W, Jin F, Song Y, Wang Y, Huo N, Chen L, Qian H, Hou R, Duan Y, Jin Y. Periapical follicle stem cell: a promising candidate for cementum/periodontal ligament regeneration and bio-root engineering. Stem Cells Dev. 2010;19(9):1405–15. https://doi.org/10.1089/scd.2009.0277 .
Luan X, Ito Y, Dangaria S, Diekwisch TG. Dental follicle progenitor cell heterogeneity in the developing mouse periodontium. Stem Cells Dev. 2006;15(4):595–608. https://doi.org/10.1089/scd.2006.15.595 .
Handa K, Saito M, Tsunoda A, Yamauchi M, Hattori S, Sato S, Toyoda M, Teranaka T, Narayanan AS. Progenitor cells from dental follicle are able to form cementum matrix in vivo. Connect Tissue Res. 2002;43(2–3):406–8 PMID: 12489190.
Guo W, Chen L, Gong K, Ding B, Duan Y, Jin Y. Heterogeneous dental follicle cells and the regeneration of complex periodontal tissues. Tissue Engineering. Part A. 2012;18(5–6):459–70 https://doi.org/10.1089/ten.tea.2011.0261 .
Bai, Yudi et al. Cementum- and periodontal ligament-like tissue formation by dental follicle cell sheets co-cultured with Hertwig’s epithelial root sheath cells. Bone. 2011, 48, Issue 6, pp. 1417–1426, https://doi.org/10.1016/j.bone.2011.02.016 .
Cordeiro MM, Dong Z, Kaneko T, Zhang Z, Miyazawa M, Shi S, et al. Dental pulp tissue engineering with stem cells from exfoliated deciduous teeth. 2008, 34, pp. 962–969.
Dobie K, Smith G, Sloan AJ, Smith AJ. Effects of alginate, hydrogels and TGF-beta 1 on human dental pulp repair in vitro. Connect Tissue Res 2. 2002;43:387–90.
Friedlander LT, Cullinan MP, Love RM. Dental stem cells and their potential role in apexogenesis and apexification. Int Endod J. 2009;42:955–62.
Cai J, Zhang Y, Liu P, Chen S, Wu X, Sun Y, Li A, Huang K, Luo R, Wang L, Liu Y, Zhou T, Wei S, Pan G, Pei D, Generation of tooth-like structures from integration-free human urine induced pluripotent stem cells. Cell Regen (Lond). July 30, 2013, 2(1), pp. 6, doi: https://doi.org/10.1186/2045-9769-2-6 .
Craig J. Taylor, Eleanor M. Bolton, and J. Andrew Bradley 2011 Aug 12 and https://doi.org/10.1098/rstb.2011.0030 ], 366(1575): 2312–2322. [doi:. Immunological considerations for embryonic and induced pluripotent stem cell banking,. Philos Trans R SocLond B Biol Sci. 2011, 366(1575), pp. 2312–2322, doi: https://doi.org/10.1098/rstb.2011.0030 .
T.R. Nayak, H. Andersen, V.S. Makam, C. Khaw, S. Bae, X.F. Xu, P.L.R. Ee, J.H. Ahn, B.H. Hong, G. Pastorin, B. Ozyilmaz, ACS Nano, 5 (6) (2011), pp. 4. Graphene for controlled and accelerated osteogenic differentiation of human mesenchymal stem cells,. ACS Nano. 2011, pp. 4670–4678.
Lee WC, Lim C, Shi H, Tang LAL, Wang Y, Lim CT, Loh KP. Origin of enhanced stem cell growth and differentiation on graphene and graphene oxide. ACS Nano. 2011;5(9):7334–41.
Kenry LWC, Loh KP, Lim CT. When stem cells meet graphene: opportunities and challenges in regenerative medicine. Biomaterials. 2018;155:236–50.
Yuan A, Farber EL, Rapoport AL, Tejada D, Deniskin R, Akhmedov NB, et al. Transfer of microRNAs by embryonic stem cell microvesicles. 2009. 2009, 4(3), p. https://doi.org/10.1371/journal.pone . 0004722.
Oh, Myeongsik, et al. Exosomes derived from human induced pluripotent stem cells ameliorate the aging of skin fibroblasts. Int. J. Mol. Sci. 2018, 19(6), p. 1715.
Ramirez MI. et al. Technical challenges of working with extracellular vesicles. Nanoscale. 2018;10:881–906.
Valadi H, et al. Exosome-mediated transfer of mRNAs and microRNAs is a novel mechanism of genetic exchange between cells. Nat. Cell Biol. 2007;9:654–9.
Mateescu B, et al. Obstacles and opportunities in the functional analysis of extracellular vesicle RNA—an ISEV position paper. J. Extracell. Vesicles. 2017;6(1). https://doi.org/10.1080/20013078.2017.1286095 .
Nawaz M, et al. Extracellular vesicles: evolving factors in stem cell biology. Stem Cells Int. 2016;2016:17. Article ID 1073140.
Helfrich, Y.R., Sachs, D.L. and Voorhees, J.J. Overview of skin aging and photoaging. Dermatol. Nurs. 20, pp. 177–183, https://www.ncbi.nlm.nih.gov/pubmed/18649702 .
Julia Tigges, Jean Krutmann, Ellen Fritsche, Judith Haendeler, Heiner Schaal, Jens W. Fischer, Faiza Kalfalah, Hans Reinke, Guido Reifenberger, Kai Stühler, Natascia Ventura, Sabrina Gundermann, Petra Boukamp, Fritz Boege. The hallmarks of fibroblast ageing, mechanisms of ageing and development, 138, 2014, Pages 26–44. 2014, 138, pp. 26–44, ISSN 0047–6374, https://doi.org/10.1016/j.mad.2014.03.004 .
Huh MI, Kim MS, Kim HK, et al. Effect of conditioned media collected from human amniotic fluid-derived stem cells (hAFSCs) on skin regeneration and photo-aging. Tissue Eng Regen Med. 2014;11:171 https://doi.org/10.1007/s13770-014-0412-1 .
Togel F, Hu Z, Weiss K, et al. Administered mesenchymal stem cells protect against ischemic acute renal failure through differentiation-independent mechanisms. Am J Physiol Renal Physiol. 2005;289:F31.
Liu J, Han G, Liu H, et al. Suppression of cholangiocarcinoma cell growth by human umbilical cord mesenchymal stem cells: a possible role of Wnt and Akt signaling. PLoS One. 2013;8:e62844.
Oh M, et al. Promotive effects of human induced pluripotent stem cell-conditioned medium on the proliferation and migration of dermal fibroblasts. Biotechnol. Bioprocess Eng. 2017;22:561–8.
Chen L, Tredget EE, Wu PY, Wu Y. Paracrine factors of mesenchymal stem cells recruit macrophages and endothelial lineage cells and enhance wound healing. PloS One. 2008;3:e1886.
Bae J-S, Lee S-H, Kim J-E, Choi J-Y, Park R-W, Park JY, Park H-S, Sohn Y-S, Lee D-S, Lee EB. βig-h3 supports keratinocyte adhesion, migration, and proliferation through α3β1 integrin. Biochem. Biophys. Res. Commun. 2002;294:940–8.
Zhou B-R, Xu Y, Guo S-L, Xu Y, Wang Y, Zhu F, Permatasari F, Wu D, Yin Z-Q, Luo D. The effect of conditioned media of adipose-derived stem cells on wound healing after ablative fractional carbon dioxide laser resurfacing. BioMed Res. Int. 2013;519:126.
Peng Y, Baulier E, Ke Y, Young A, Ahmedli NB, Schwartz SD, et al. Human embryonic stem cells extracellular vesicles and their effects on immortalized human retinal Müller cells. PLoS ONE. 2018, 13(3), p. https://doi.org/10.1371/journal.pone.019400 .
Harris MT, Butler DL, Boivin GP, Florer JB, Schantz EJ, Wenstrup RJ. Mesenchymal stem cells used for rabbit tendon repair can form ectopic bone and express alkaline phosphatase activity in constructs. J Orthop Res. 2004;22:998–1003.
Mascetti VL, Pedersen RA. Human-mouse chimerism validates human stem cell pluripotency. Cell Stem Cell. 2016;18:67–72.
Gandia C, Armiñan A, García-Verdugo JM, Lledó E, Ruiz A, Miñana MD, Sanchez-Torrijos J, Payá R, Mirabet V, Carbonell-Uberos F, Llop M, Montero JA, Sepúlveda P. Human dental pulp stem cells improve left ventricular function, induce angiogenesis, and reduce infarct size in rats with acute myocardial infarction. Stem Cells. 2007;26(3):638–45.
Perry BC, Zhou D, Wu X, Yang FC, Byers MA, Chu TM, Hockema JJ, Woods EJ, Goebel WS. Collection, cryopreservation, and characterization of human dental pulp-derived mesenchymal stem cells for banking and clinical use. Tissue Eng Part C Methods. 2008;14(2):149–56.
Garcia-Olmo D, Garcia-Arranz M, Herreros D, et al. A phase I clinical trial of the treatment of Crohn’s fistula by adipose mesenchymal stem cell transplantation. Dis Colon Rectum. 2005;48:1416–23.
de Mendonça CA, Bueno DF, Martins MT, Kerkis I, Kerkis A, Fanganiello RD, Cerruti H, Alonso N, Passos-Bueno MR. Reconstruction of large cranial defects in nonimmunosuppressed experimental design with human dental pulp stem cells. J Craniofac Surg. 2008;19(1):204–10.
Seo BM, Sonoyama W, Yamaza T, Coppe C, Kikuiri T, Akiyama K, Lee JS, Shi S. SHED repair critical-size calvarial defects in mice. Oral Dis. 2008;14(5):428–34.
Abbas, Diakonov I., Sharpe P. Neural crest origin of dental stem cells. Pan European Federation of the International Association for Dental Research (PEF IADR). 2008, Vols. Seq #96 - Oral Stem Cells.
Kerkis I, Ambrosio CE, Kerkis A, Martins DS, Gaiad TP, Morini AC, Vieira NM, Marina P, et al. Early transplantation of human immature dental pulp stem cells from baby teeth to golden retriever muscular dystrophy (GRMD) dogs. J Transl Med. 2008;6:35.
Xianrui Yang, Li Li, Li Xiao, Donghui Zhang. Recycle the dental fairy’s package: overview of dental pulp stem cells. Stem Cell Res Ther . 2018, 9, 1, 1. https://doi.org/10.1186/s13287-018-1094-8 .
Wang J, Wang X, Sun Z, Wang X, Yang H, Shi S, Wang S. Stem cells from human-exfoliated deciduous teeth can differentiate into dopaminergic neuron-like cells. Stem Cells Dev. 2010;19:1375–83.
Wang J, et al. The odontogenic differentiation of human dental pulp stem cells on nanofibrous poly (L-lactic acid) scaffolds in vitro and in vivo. Acta Biomater. 2010;6(10):3856–63.
Davies OG, Cooper PR, Shelton RM, Smith AJ, Scheven BA. A comparison of the in vitro mineralisation and dentinogenic potential of mesenchymal stem cells derived from adipose tissue, bone marrow and dental pulp. J Bone Miner Metab. 2015;33:371–82.
Huang GT-J, Shagramanova K, Chan SW. Formation of odontoblast-like cells from cultured human dental pulp cells on dentin in vitro. J Endod. 2006;32:1066–73.
Shi S, Robey PG, Gronthos S. Comparison of human dental pulp and bone marrow stromal stem cells by cDNA microarray analysis. Bone. 2001;29(6):532–9.
Gronthos S, Mankani M, Brahim J, Robey PG, Shi S. Postnatal human dental pulp stem cells (DPSCs) in vitro and in vivo. Proc Natl Acad Sci U S A. 2000;97:13625–30.
Nuti N, Corallo C, Chan BMF, Ferrari M, Gerami-Naini B. Multipotent differentiation of human dental pulp stem cells: a literature review. Stem Cell Rev Rep. 2016;12:511–23.
Ferro F, et al. Dental pulp stem cells differentiation reveals new insights in Oct4A dynamics. PloS One. 2012;7(7):e41774.
Conde MCM, Chisini LA, Grazioli G, Francia A, Carvalho RVd, Alcázar JCB, Tarquinio SBC, Demarco FF. Does cryopreservation affect the biological properties of stem cells from dental tissues? A systematic review. Braz Dent J. 2016;1210(6):633-40. https://doi.org/10.1590/0103-6440201600980 .
Papaccio G, Graziano A, d’Aquino R, Graziano MF, Pirozzi G, Menditti D, De Rosa A, Carinci F, Laino G. Long-term cryopreservation of dental pulp stem cells (SBP-DPSCs) and their differentiated osteoblasts: a cell source for tissue repair. J Cell Physiol. 2006;208:319–25.
Alge DL, Zhou D, Adams LL, et. al. Donor-matched comparison of dental pulp stem cells and bone marrow-derived mesenchymal stem cells in a rat model. J Tissue Eng Regen Med. 2010;4(1):73–81.
Jo Y-Y, Lee H-J, Kook S-Y, Choung H-W, Park J-Y, Chung J-H, Choung Y-H, Kim E-S, Yang H-C, Choung P-H. Isolation and characterization of postnatal stem cells from human dental tissues. Tissue Eng. 2007;13:767–73.
Gronthos S, Brahim J, Li W, Fisher LW, Cherman N, Boyde A, DenBesten P, Robey PG, Shi S. Stem cell properties of human dental pulp stem cells. J Dent Res. 2002;81:531–5.
Laino G, d’Aquino R, Graziano A, Lanza V, Carinci F, Naro F, Pirozzi G, Papaccio G. A new population of human adult dental pulp stem cells: a useful source of living autologous fibrous bone tissue (LAB). J Bone Miner Res. 2005;20:1394–402.
Zainal A, Shahrul H, et al. In vitro chondrogenesis transformation study of mouse dental pulp stem cells. Sci World J. 2012;2012:827149.
Wei X, et al. Expression of mineralization markers in dental pulp cells. J Endod. 2007;33(6):703–8.
Dai J, et al. The effect of co-culturing costal chondrocytes and dental pulp stem cells combined with exogenous FGF9 protein on chondrogenesis and ossification in engineered cartilage. Biomaterials. 2012;33(31):7699–711.
Vasandan AB, et al. Functional differences in mesenchymal stromal cells from human dental pulp and periodontal ligament. J Cell Mol Med. 2014;18(2):344–54.
Werle SB, et al. Carious deciduous teeth are a potential source for dental pulp stem cells. Clin Oral Investig. 2015;20:75–81.
Nemeth CL, et al. Enhanced chondrogenic differentiation of dental pulp stem cells using nanopatterned PEG-GelMA-HA hydrogels. Tissue Eng A. 2014;20(21–22):2817–29.
Paino F, Ricci G, De Rosa A, D’Aquino R, Laino L, Pirozzi G, et al. Ecto-mesenchymal stem cells from dental pulp are committed to differentiate into active melanocytes. Eur. Cell Mater. 2010;20:295–305.
Ferro F, Spelat R, Baheney CS. Dental pulp stem cell (DPSC) isolation, characterization, and differentiation. In: Kioussi C, editor. Stem cells and tissue repair. Methods in molecular biology (methods and protocols): Humana Press. 2014;1210.
Ishkitiev N, Yaegaki K, Imai T, Tanaka T, Nakahara T, Ishikawa H, Mitev V, Haapasalo M. High-purity hepatic lineage differentiated from dental pulp stem cells in serum-free medium. J Endod. 2012;38:475–80.
Download references
Acknowledgements
Not applicable.
This work is supported by Wrocław Medical University in Poland.
Availability of data and materials
Please contact author for data requests.
Author information
Authors and affiliations.
Department of Experimental Surgery and Biomaterials Research, Wroclaw Medical University, Bujwida 44, Wrocław, 50-345, Poland
Wojciech Zakrzewski, Maria Szymonowicz & Zbigniew Rybak
Department of Conservative Dentistry and Pedodontics, Krakowska 26, Wrocław, 50-425, Poland
Maciej Dobrzyński
You can also search for this author in PubMed Google Scholar
Contributions
WZ is the principal author and was responsible for the first draft of the manuscript. WZ and ZR were responsible for the concept of the review. MS, MD, and ZR were responsible for revising the article and for data acquisition. All authors read and approved the final manuscript.
Corresponding author
Correspondence to Wojciech Zakrzewski .
Ethics declarations
Ethics approval and consent to participate, consent for publication, competing interests.
The authors declare that they have no competing interests.
Publisher’s Note
Springer Nature remains neutral with regard to jurisdictional claims in published maps and institutional affiliations.
Rights and permissions
Open Access This article is distributed under the terms of the Creative Commons Attribution 4.0 International License ( http://creativecommons.org/licenses/by/4.0/ ), which permits unrestricted use, distribution, and reproduction in any medium, provided you give appropriate credit to the original author(s) and the source, provide a link to the Creative Commons license, and indicate if changes were made. The Creative Commons Public Domain Dedication waiver ( http://creativecommons.org/publicdomain/zero/1.0/ ) applies to the data made available in this article, unless otherwise stated.
Reprints and permissions
About this article
Cite this article.
Zakrzewski, W., Dobrzyński, M., Szymonowicz, M. et al. Stem cells: past, present, and future. Stem Cell Res Ther 10 , 68 (2019). https://doi.org/10.1186/s13287-019-1165-5
Download citation
Published : 26 February 2019
DOI : https://doi.org/10.1186/s13287-019-1165-5
Share this article
Anyone you share the following link with will be able to read this content:
Sorry, a shareable link is not currently available for this article.
Provided by the Springer Nature SharedIt content-sharing initiative
- Differentiation
- Pluripotency
- Induced pluripotent stem cell (iPSC)
- Stem cell derivation
- Growth media
- Tissue banks
- Tissue transplantation
Stem Cell Research & Therapy
ISSN: 1757-6512
- Submission enquiries: Access here and click Contact Us
- General enquiries: [email protected]
Stem cells: past, present, and future
Affiliations.
- 1 Department of Experimental Surgery and Biomaterials Research, Wroclaw Medical University, Bujwida 44, Wrocław, 50-345, Poland. [email protected].
- 2 Department of Conservative Dentistry and Pedodontics, Krakowska 26, Wrocław, 50-425, Poland.
- 3 Department of Experimental Surgery and Biomaterials Research, Wroclaw Medical University, Bujwida 44, Wrocław, 50-345, Poland.
- PMID: 30808416
- PMCID: PMC6390367
- DOI: 10.1186/s13287-019-1165-5
In recent years, stem cell therapy has become a very promising and advanced scientific research topic. The development of treatment methods has evoked great expectations. This paper is a review focused on the discovery of different stem cells and the potential therapies based on these cells. The genesis of stem cells is followed by laboratory steps of controlled stem cell culturing and derivation. Quality control and teratoma formation assays are important procedures in assessing the properties of the stem cells tested. Derivation methods and the utilization of culturing media are crucial to set proper environmental conditions for controlled differentiation. Among many types of stem tissue applications, the use of graphene scaffolds and the potential of extracellular vesicle-based therapies require attention due to their versatility. The review is summarized by challenges that stem cell therapy must overcome to be accepted worldwide. A wide variety of possibilities makes this cutting edge therapy a turning point in modern medicine, providing hope for untreatable diseases.
Keywords: Differentiation; Growth media; Induced pluripotent stem cell (iPSC); Pluripotency; Stem cell derivation; Stem cells; Teratoma formation assay; Tissue banks; Tissue transplantation.
Publication types
- Research Support, Non-U.S. Gov't
- Cell Differentiation / genetics*
- Cell- and Tissue-Based Therapy / trends*
- Graphite / chemistry
- Graphite / therapeutic use
- Induced Pluripotent Stem Cells / transplantation*
- Stem Cell Transplantation / classification
- Stem Cells / classification
- Stem Cells / cytology*
- Tissue Scaffolds / chemistry
Appointments at Mayo Clinic
Stem cells: what they are and what they do.
Stem cells offer promise for new medical treatments. Learn about stem cell types, current and possible uses, and the state of research and practice.
You've heard about stem cells in the news, and perhaps you've wondered if they might help you or a loved one with a serious disease. Here are some answers to frequently asked questions about stem cells.
What are stem cells?
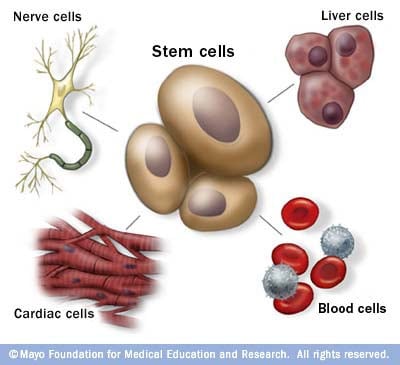
Stem cells: The body's master cells
Stem cells are the body's master cells. All other cells arise from stem cells, including blood cells, nerve cells and other cells.
Stem cells are a special type of cells that have two important properties. They are able to make more cells like themselves. That is, they self-renew. And they can become other cells that do different things in a process known as differentiation. Stem cells are found in almost all tissues of the body. And they are needed for the maintenance of tissue as well as for repair after injury.
Depending on where the stem cells are, they can develop into different tissues. For example, hematopoietic stem cells reside in the bone marrow and can produce all the cells that function in the blood. Stem cells also can become brain cells, heart muscle cells, bone cells or other cell types.
There are various types of stem cells. Embryonic stem cells are the most versatile since they can develop into all the cells of the developing fetus. The majority of stem cells in the body have fewer abilities to give rise to cells and may only help maintain and repair the tissues and organs in which they reside.
No other cell in the body has the natural ability to generate new cell types.
Why is there such an interest in stem cells?
Researchers are studying stem cells to see if they can help to:
- Increase understanding of how diseases occur. By watching stem cells mature into cells in bones, heart muscle, nerves, and other organs and tissue, researchers may better understand how diseases and conditions develop.
Generate healthy cells to replace cells affected by disease (regenerative medicine). Stem cells can be guided into becoming specific cells that can be used in people to regenerate and repair tissues that have been damaged or affected by disease.
People who might benefit from stem cell therapies include those with leukemia, Hodgkin disease, non-Hodgkin lymphoma and some solid tumor cancers. Stem cell therapies also might benefit people who have aplastic anemia, immunodeficiencies and inherited conditions of metabolism.
Stem cells are being studied to treat type 1 diabetes, Parkinson's disease, amyotrophic lateral sclerosis, heart failure, osteoarthritis and other conditions.
Stem cells may have the potential to be grown to become new tissue for use in transplant and regenerative medicine. Researchers continue to advance the knowledge on stem cells and their applications in transplant and regenerative medicine.
Test new drugs for safety and effectiveness. Before giving drugs in development to people, researchers can use some types of stem cells to test the drugs for safety and quality. This type of testing may help assess drugs in development for toxicity to the heart.
New areas of study include the effectiveness of using human stem cells that have been programmed into tissue-specific cells to test new drugs. For the testing of new drugs to be accurate, the cells must be programmed to acquire properties of the type of cells targeted by the drug. Techniques to program cells into specific cells are under study.
Where do stem cells come from?
There are several sources of stem cells:
Embryonic stem cells. These stem cells come from embryos that are 3 to 5 days old. At this stage, an embryo is called a blastocyst and has about 150 cells.
These are pluripotent (ploo-RIP-uh-tunt) stem cells, meaning they can divide into more stem cells or can become any type of cell in the body. This allows embryonic stem cells to be used to regenerate or repair diseased tissue and organs.
- Adult stem cells. These stem cells are found in small numbers in most adult tissues, such as bone marrow or fat. Compared with embryonic stem cells, adult stem cells have a more limited ability to give rise to various cells of the body.
Adult cells altered to have properties of embryonic stem cells. Scientists have transformed regular adult cells into stem cells using genetic reprogramming. By altering the genes in the adult cells, researchers can make the cells act similarly to embryonic stem cells. These cells are called induced pluripotent stem cells (iPSCs).
This new technique may allow use of reprogrammed cells instead of embryonic stem cells and prevent immune system rejection of the new stem cells. However, scientists don't yet know whether using altered adult cells will cause adverse effects in humans.
Researchers have been able to take regular connective tissue cells and reprogram them to become functional heart cells. In studies, animals with heart failure that were injected with new heart cells had better heart function and survival time.
Perinatal stem cells. Researchers have discovered stem cells in amniotic fluid as well as umbilical cord blood. These stem cells can change into specialized cells.
Amniotic fluid fills the sac that surrounds and protects a developing fetus in the uterus. Researchers have identified stem cells in samples of amniotic fluid drawn from pregnant women for testing or treatment — a procedure called amniocentesis.
Why is there controversy about using embryonic stem cells?
The National Institutes of Health created guidelines for human stem cell research in 2009. The guidelines define embryonic stem cells and how they may be used in research and include recommendations for the donation of embryonic stem cells. Also, the guidelines state that embryonic stem cells from embryos created by in vitro fertilization can be used only when the embryo is no longer needed.
Where do these embryos come from?
The embryos being used in embryonic stem cell research come from eggs that were fertilized at in vitro fertilization clinics but never implanted in women's uteruses. The stem cells are donated with informed consent from donors. The stem cells can live and grow in special solutions in test tubes or petri dishes in laboratories.
Why can't researchers use adult stem cells instead?
Progress in cell reprogramming and the formation of iPSCs has greatly enhanced research in this field. However, reprogramming is an inefficient process. When possible, iPSCs are used instead of embryonic stem cells since this avoids the ethical issues about use of embryonic stem cells that may be morally objectionable for some people.
Although research into adult stem cells is promising, adult stem cells may not be as versatile and durable as are embryonic stem cells. Adult stem cells may not be able to be manipulated to produce all cell types, which limits how adult stem cells can be used to treat diseases.
Adult stem cells are also more likely to contain irregularities due to environmental hazards, such as toxins, or from errors acquired by the cells during replication. However, researchers have found that adult stem cells are more adaptable than was first thought.
What are stem cell lines, and why do researchers want to use them?
A stem cell line is a group of cells that all descend from a single original stem cell and are grown in a lab. Cells in a stem cell line keep growing but don't become specialized cells. Ideally, they remain free of genetic defects and continue to create more stem cells. Clusters of cells can be taken from a stem cell line and frozen for storage or shared with other researchers.
What is stem cell therapy (regenerative medicine), and how does it work?
Stem cell therapy, also known as regenerative medicine, promotes the repair response of diseased, dysfunctional or injured tissue using stem cells or their derivatives. It is the next chapter in organ transplantation and uses cells instead of donor organs, which are limited in supply.
Researchers grow stem cells in a lab. These stem cells are manipulated to specialize into specific types of cells, such as heart muscle cells, blood cells or nerve cells.
The specialized cells can then be implanted into a person. For example, if the person has heart disease, the cells could be injected into the heart muscle. The healthy transplanted heart muscle cells could then contribute to repairing the injured heart muscle.
Researchers have already shown that adult bone marrow cells guided to become heart-like cells can repair heart tissue in people, and more research is ongoing.
Have stem cells already been used to treat diseases?
Yes. Doctors have performed stem cell transplants, also known as bone marrow transplants, for many decades. In hematopoietic stem cell transplants, stem cells replace cells damaged by chemotherapy or disease or serve as a way for the donor's immune system to fight some types of cancer and blood-related diseases. Leukemia, lymphoma, neuroblastoma and multiple myeloma often are treated this way. These transplants use adult stem cells or umbilical cord blood.
Researchers are testing adult stem cells to treat other conditions, including some degenerative diseases such as heart failure.
What are the potential problems with using embryonic stem cells in humans?
For embryonic stem cells to be useful, researchers must be certain that the stem cells will differentiate into the specific cell types desired.
Researchers have discovered ways to direct stem cells to become specific types of cells, such as directing embryonic stem cells to become heart cells. Research is ongoing in this area.
Embryonic stem cells also can grow irregularly or specialize in different cell types spontaneously. Researchers are studying how to control the growth and development of embryonic stem cells.
Embryonic stem cells also might trigger an immune response in which the recipient's body attacks the stem cells as foreign invaders, or the stem cells might simply fail to function as expected, with unknown consequences. Researchers continue to study how to avoid these possible complications.
What is therapeutic cloning, and what benefits might it offer?
Therapeutic cloning, also called somatic cell nuclear transfer, is a way to create versatile stem cells independent of fertilized eggs. In this technique, the nucleus is removed from an unfertilized egg. This nucleus contains the genetic material. The nucleus also is removed from the cell of a donor.
This donor nucleus is then injected into the egg, replacing the nucleus that was removed, in a process called nuclear transfer. The egg is allowed to divide and soon forms a blastocyst. This process creates a line of stem cells that is genetically identical to the donor's cells — in essence, a clone.
Some researchers believe that stem cells derived from therapeutic cloning may offer benefits over those from fertilized eggs because cloned cells are less likely to be rejected once transplanted back into the donor. And it may allow researchers to see exactly how a disease develops.
Has therapeutic cloning in people been successful?
No. Researchers haven't been able to successfully perform therapeutic cloning with humans despite success in a number of other species.
Researchers continue to study the potential of therapeutic cloning in people.
There is a problem with information submitted for this request. Review/update the information highlighted below and resubmit the form.
From Mayo Clinic to your inbox
Sign up for free and stay up to date on research advancements, health tips, current health topics, and expertise on managing health. Click here for an email preview.
Error Email field is required
Error Include a valid email address
To provide you with the most relevant and helpful information, and understand which information is beneficial, we may combine your email and website usage information with other information we have about you. If you are a Mayo Clinic patient, this could include protected health information. If we combine this information with your protected health information, we will treat all of that information as protected health information and will only use or disclose that information as set forth in our notice of privacy practices. You may opt-out of email communications at any time by clicking on the unsubscribe link in the e-mail.
Thank you for subscribing!
You'll soon start receiving the latest Mayo Clinic health information you requested in your inbox.
Sorry something went wrong with your subscription
Please, try again in a couple of minutes
- Stem cell basics. National Institutes of Health. https://stemcells.nih.gov/info/basics/stc-basics/#stc-I. Accessed March 21, 2024.
- Lovell-Badge R, et al. ISSCR guidelines for stem cell research and clinical translation: The 2021 update. Stem Cell Reports. 2021; doi:10.1016/j.stemcr.2021.05.012.
- AskMayoExpert. Hematopoietic stem cell transplant. Mayo Clinic; 2024.
- Stem cell transplants in cancer treatment. National Cancer Institute. https://www.cancer.gov/about-cancer/treatment/types/stem-cell-transplant/. Accessed March 21, 2024.
- Townsend CM Jr, et al. Regenerative medicine. In: Sabiston Textbook of Surgery: The Biological Basis of Modern Surgical Practice. 21st ed. Elsevier; 2022. https://www.clinicalkey.com. Accessed March 21, 2024.
- Kumar D, et al. Stem cell based preclinical drug development and toxicity prediction. Current Pharmaceutical Design. 2021; doi:10.2174/1381612826666201019104712.
- NIH guidelines for human stem cell research. National Institutes of Health. https://stemcells.nih.gov/research-policy/guidelines-for-human-stem-cell-research. Accessed March 21, 2024.
- De la Torre P, et al. Current status and future prospects of perinatal stem cells. Genes. 2020; doi:10.3390/genes12010006.
- Yen Ling Wang A. Human induced pluripotent stem cell-derived exosomes as a new therapeutic strategy for various diseases. International Journal of Molecular Sciences. 2021; doi:10.3390/ijms22041769.
- Alessandrini M, et al. Stem cell therapy for neurological disorders. South African Medical Journal. 2019; doi:10.7196/SAMJ.2019.v109i8b.14009.
- Goldenberg D, et al. Regenerative engineering: Current applications and future perspectives. Frontiers in Surgery. 2021; doi:10.3389/fsurg.2021.731031.
- Brown MA, et al. Update on stem cell technologies in congenital heart disease. Journal of Cardiac Surgery. 2020; doi:10.1111/jocs.14312.
- Li M, et al. Brachyury engineers cardiac repair competent stem cells. Stem Cells Translational Medicine. 2021; doi:10.1002/sctm.20-0193.
- Augustine R, et al. Stem cell-based approaches in cardiac tissue engineering: Controlling the microenvironment for autologous cells. Biomedical Pharmacotherapy. 2021; doi:10.1016/j.biopha.2021.111425.
- Cloning fact sheet. National Human Genome Research Institute. https://www.genome.gov/about-genomics/fact-sheets/Cloning-Fact-Sheet. Accessed March 21, 2024.
- Dingli D (expert opinion). Mayo Clinic. Nov. 17, 2023.
Products and Services
- A Book: Living Medicine
- Sign up for Email: Get Your Free Resource – Coping with Cancer
- Give today to find cancer cures for tomorrow
- Acute lymphocytic leukemia
- Acute myelogenous leukemia
- Adjuvant therapy for cancer
- Amyloidosis
- Aplastic anemia
- Atypical cells: Are they cancer?
- Biopsy procedures
- Blood Cancers and Disorders
- Bone marrow transplant
- Cancer blood tests
- Myths about cancer causes
- Infographic: Cancer Clinical Trials Offer Many Benefits
- Cancer diagnosis: 11 tips for coping
- Cancer-related fatigue
- Cancer pain: Relief is possible
- Cancer risk: What the numbers mean
- Cancer surgery
- Cancer survival rate
- Cancer survivors: Care for your body after treatment
- Cancer survivors: Late effects of cancer treatment
- Cancer survivors: Managing your emotions after cancer treatment
- Cancer treatment myths
- Chemotherapy side effects: A cause of heart disease?
- Chronic lymphocytic leukemia
- Chronic myelogenous leukemia
- Curcumin: Can it slow cancer growth?
- What is type 1 diabetes? A Mayo Clinic expert explains
- Type 1 diabetes FAQs
- Cancer-related diarrhea
- DiGeorge syndrome (22q11.2 deletion syndrome)
- Eating during cancer treatment: Tips to make food tastier
- Epidermolysis bullosa
- Gaucher disease
- Heart cancer: Is there such a thing?
- High-dose vitamin C: Can it kill cancer cells?
- Hodgkin's lymphoma (Hodgkin's disease)
- Hodgkin's vs. non-Hodgkin's lymphoma: What's the difference?
- Low blood counts
- Measles Virus as a Cancer Fighter
- Monoclonal antibody drugs
- Mort Crim and Cancer
- Mouth sores caused by cancer treatment: How to cope
- Multiple myeloma
- Infographic: Multiple Myeloma
- Myelofibrosis
- Neuroblastoma
- No appetite? How to get nutrition during cancer treatment
- Non-Hodgkin's lymphoma
- Scleroderma
- Self-Image During Cancer
- Sickle cell anemia
- Sisters' Bone Marrow Transplant
- Small cell, large cell cancer: What this means
- Stem Cells 101
- Thalassemia
- Tumor vs. cyst: What's the difference?
- Type 1 diabetes
- Stem cell transplant
- How cancer spreads
- PICC line placement
- When cancer returns: How to cope with cancer recurrence
Mayo Clinic does not endorse companies or products. Advertising revenue supports our not-for-profit mission.
- Opportunities
Mayo Clinic Press
Check out these best-sellers and special offers on books and newsletters from Mayo Clinic Press .
- Mayo Clinic on Incontinence - Mayo Clinic Press Mayo Clinic on Incontinence
- The Essential Diabetes Book - Mayo Clinic Press The Essential Diabetes Book
- Mayo Clinic on Hearing and Balance - Mayo Clinic Press Mayo Clinic on Hearing and Balance
- FREE Mayo Clinic Diet Assessment - Mayo Clinic Press FREE Mayo Clinic Diet Assessment
- Mayo Clinic Health Letter - FREE book - Mayo Clinic Press Mayo Clinic Health Letter - FREE book
- Stem cells What they are and what they do
Make twice the impact
Your gift can go twice as far to advance cancer research and care!
- Search Menu
- Advance Articles
- Author Guidelines
- Submission Site
- Open Access Options
- Self-Archiving Policy
Call for Papers
- Why Publish with Us?
- Advertising & Corporate Services
- Reprints, ePrints, Supplements
- About STEM CELLS
- Editorial Board
- Reviewer Guidelines
- Journals on Oxford Academic
- Books on Oxford Academic

STEM CELLS , a peer-reviewed journal published monthly, provides a forum for prompt publication of original investigative papers and concise reviews. STEM CELLS is read and written by clinical and basic scientists whose expertise encompasses the rapidly expanding fields of stem and progenitor cell biology.
Discover the benefits of publishing with us.
Instructions to authors
When submitting your manuscript, please indicate your interest in the call for papers in your cover letter, and select the appropriate topic in the “Call for Papers” jump menu where prompted on the title page information screen.
Clicking on a Call for Papers in the table above will take you to more information about that topic.
Mesenchymal Stem/stromal Cells (MSCs) or exosomes for disease or injury modification
There is discussion in the stem cell field about what product will be best for treating different disease indications- MSCs or their exosomes? There are pros and cons to each product, and one might be better than the other for the treatment of specific diseases or tissue injuries. For instance, exosomes might replace the functions of IV-administered MSCs for treating Graft vs. Host disease or for other immunomodulatory functions, whereas for tissue or bone repair MSCs injected locally and engineered to be retained for longer periods of time might be best, to orchestrate healing.
Submit your article
- Recommend to Your Librarian
- Advertising and Corporate Services
Affiliations

- Online ISSN 1549-4918
- Copyright © 2024 Oxford University Press
- About Oxford Academic
- Publish journals with us
- University press partners
- What we publish
- New features
- Open access
- Institutional account management
- Rights and permissions
- Get help with access
- Accessibility
- Advertising
- Media enquiries
- Oxford University Press
- Oxford Languages
- University of Oxford
Oxford University Press is a department of the University of Oxford. It furthers the University's objective of excellence in research, scholarship, and education by publishing worldwide
- Copyright © 2024 Oxford University Press
- Cookie settings
- Cookie policy
- Privacy policy
- Legal notice
This Feature Is Available To Subscribers Only
Sign In or Create an Account
This PDF is available to Subscribers Only
For full access to this pdf, sign in to an existing account, or purchase an annual subscription.
BMSC-derived Exosomes Ameliorate Peritoneal Dialysis-associated Peritoneal Fibrosis via the Mir-27a-3p/TP53 Pathway
- Published: 16 April 2024
Cite this article
- Jun-li Zhao 1 na1 ,
- Lin Zhao 2 na1 ,
- Qiu-nan Zhan 1 ,
- Miao Liu 1 ,
- Ting Zhang 1 &
- Wen-wen Chu 1
20 Accesses
Explore all metrics
Peritoneal fibrosis (PF) is the main cause of declining efficiency and ultrafiltration failure of the peritoneum, which restricts the long-term application of peritoneal dialysis (PD). This study aimed to investigate the therapeutic effects and mechanisms of bone marrow mesenchymal stem cells-derived exosomes (BMSC-Exos) on PF in response to PD.
Small RNA sequencing analysis of BMSC-Exos was performed by second-generation sequencing. C57BL/6J mice were infused with 4.25% glucose-based peritoneal dialysis fluid (PDF) for 6 consecutive weeks to establish a PF model. A total of 36 mice were randomly divided into 6 groups: control group, 1.5% PDF group, 2.5% PDF group, 4.25% PDF group, BMSC-Exos treatment group, and BMSC-Exos+TP53 treatment group. Reverse transcription quantitative polymerase chain reaction (RT-qPCR) was performed to measure the expression level of miR-27a-3p in BMSC-Exos and peritoneum of mice treated with different concentrations of PDF. HE and Masson staining were performed to evaluate the extent of PF. The therapeutic potential of BMSC-Exos for PF was examined through pathological examination, RT-qPCR, Western blotting, and peritoneal function analyses. Epithelial-mesenchymal transition (EMT) of HMrSV5 was induced with 4.25% PDF. Cells were divided into control group, 4.25% PDF group, BMSC-Exos treatment group, and BMSC-Exos+TP53 treatment group. Cell Counting Kit-8 assay was used to measure cell viability, and transwell migration assay was used to verify the capacity of BMSC-Exos to inhibit EMT in HMrSV5 cells.
Small RNA sequencing analysis showed that miR-27a-3p was highly expressed in BMSC-derived exosomes compared to BMSCs. The RT-qPCR results showed that the expression of miR-27a-3p was upregulated in BMSC-Exos, but decreased in PD mice. We found that PF was glucose concentration-dependently enhanced in the peritoneum of the PD mice. Compared with the control mice, the PD mice showed high solute transport and decreased ultrafiltration volume as well as an obvious fibroproliferative response, with markedly increased peritoneal thickness and higher expression of α-SMA, collagen-I, fibronectin, and ECM1. The mice with PD showed decreased miR-27a-3p. Peritoneal structural and functional damage was significantly attenuated after BMSC-Exos treatment, while PF and mesothelial damage were significantly ameliorated. Additionally, markers of fibrosis (α-SMA, collagen-I, fibronectin, ECM1) and profibrotic cytokines (TGF-β1, PDGF) were downregulated at the mRNA and protein levels after BMSC-Exos treatment. In HMrSV5 cells, BMSC-Exos reversed the decrease in cell viability and the increase in cell migratory capacity caused by high-glucose PDF. Western blotting and RT-qPCR analysis revealed that BMSC-Exos treatment resulted in increased expression of E-cadherin (epithelial marker) and decreased expression of α-SMA, Snail, and vimentin (mesenchymal markers) compared to those of the 4.25% PDF-treated cells. Importantly, a dual-luciferase reporter assay showed that TP53 was a target gene of miR-27a-3p. TP53 overexpression significantly reversed the decreases in PF and EMT progression induced by BMSC-Exos.
The present results demonstrate that BMSC-Exos showed an obvious protective effect on PD-related PF and suggest that BMSC-derived exosomal miR-27a-3p may exert its inhibitory effect on PF and EMT progression by targeting TP53.
This is a preview of subscription content, log in via an institution to check access.
Access this article
Price includes VAT (Russian Federation)
Instant access to the full article PDF.
Rent this article via DeepDyve
Institutional subscriptions
Similar content being viewed by others
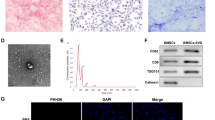
Human bone marrow mesenchymal stem cell-derived extracellular vesicles reduce inflammation and pyroptosis in acute kidney injury via miR-223-3p/HDAC2/SNRK
Zhijuan Xie, Jun Tang, … Qin Liu
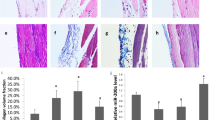
MiR-200a ameliorates peritoneal fibrosis and functional deterioration in a rat model of peritoneal dialysis
Xin Wei, Yi Bao, … Qinkai Chen
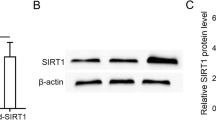
SIRT1-modified human umbilical cord mesenchymal stem cells ameliorate experimental peritoneal fibrosis by inhibiting the TGF-β/Smad3 pathway
Yanhong Guo, Liuwei Wang, … Lin Tang
Liyanage T, Ninomiya T, Jha V, et al. Worldwide access to treatment for end-stage kidney disease: a systematic review. Lancet, 2015,385(9981):1975–1982
Article PubMed Google Scholar
Cho Y, Bello AK, Levin A, et al. Peritoneal Dialysis Use and Practice Patterns: An International Survey Study. Am J Kidney Dis, 2021,77(3):315–325
Holmes CJ, Faict D. Peritoneal dialysis solution biocompatibility: definitions and evaluation strategies. Kidney Int Suppl, 2003,(88):S50-S56
Krediet RT, Struijk DG. Peritoneal changes in patients on long-term peritoneal dialysis. Nat Rev Nephrol, 2013,9(7):419–429
Article CAS PubMed Google Scholar
Teitelbaum I. Ultrafiltration failure in peritoneal dialysis: a pathophysiologic approach. Blood Purif, 2015,39(1–3):70–73
Jagirdar RM, Bozikas A, Zarogiannis SG, et al. Encapsulating Peritoneal Sclerosis: Pathophysiology and Current Treatment Options. Int J Mol Sci, 2019,20(22):5765
Article CAS PubMed PubMed Central Google Scholar
Fan YP, Hsia CC, Tseng KW, et al. The Therapeutic Potential of Human Umbilical Mesenchymal Stem Cells From Wharton’s Jelly in the Treatment of Rat Peritoneal Dialysis-Induced Fibrosis. Stem Cells Transl Med, 2016,5(2):235–247
Ueno T, Nakashima A, Doi S, et al. Mesenchymal stem cells ameliorate experimental peritoneal fibrosis by suppressing inflammation and inhibiting TGF-beta1 signaling. Kidney Int, 2013,84(2):297–307
Nagasaki K, Nakashima A, Tamura R, et al. Mesenchymal stem cells cultured in serum-free medium ameliorate experimental peritoneal fibrosis. Stem Cell Res Ther, 2021,12(1):203
Wang J, Wang L, Xu L, et al. Targeting Src attenuates peritoneal fibrosis and inhibits the epithelial to mesenchymal transition. Oncotarget, 2017,8(48):83872–83889
Article PubMed PubMed Central Google Scholar
Morishita Y, Ookawara S, Hirahara I, et al. HIF-1alpha mediates Hypoxia-induced epithelial-mesenchymal transition in peritoneal mesothelial cells. Ren Fail, 2016,38(2):282–289
Chen JS, Wong VW, Gurtner GC. Therapeutic potential of bone marrow-derived mesenchymal stem cells for cutaneous wound healing. Front Immunol, 2012,3:192
Han Y, Li X, Zhang Y, et al. Mesenchymal Stem Cells for Regenerative Medicine. Cells, 2019,8(8):886
Nagaishi K, Mizue Y, Chikenji T, et al. Mesenchymal stem cell therapy ameliorates diabetic nephropathy via the paracrine effect of renal trophic factors including exosomes. Sci Rep, 2016,6:34842
Li M, Li S, Du C, et al. Exosomes from different cells: Characteristics, modifications, and therapeutic applications. Eur J Med Chem, 2020,207:112784
Murray L, Krasnodembskaya AD. Concise Review: Intercellular Communication Via Organelle Transfer in the Biology and Therapeutic Applications of Stem Cells. Stem Cells, 2019,37(1):14–25
Fan C, Wang Q, Chen Y, et al. Exosomes derived from bone mesenchymal stem cells attenuate myocardial fibrosis both in vivo and in vitro via autophagy activation: the key role of miR-199a-3p/mTOR pathway. Hum Cell, 2022,35(3):817–835
Ma J, Li Y, Chen M, et al. hMSCs-derived exosome circCDK13 inhibits liver fibrosis by regulating the expression of MFGE8 through miR-17-5p/KAT2B. Cell Biol Toxicol, 2023,39(2):1–22
Sun C, Shi C, Duan X, et al. Exosomal microRNA-618 derived from mesenchymal stem cells attenuate the progression of hepatic fibrosis by targeting Smad4. Bioengineered, 2022,13(3):5915–5927
Xu L, Fan Y, Wu L, et al. Exosomes from Bone Marrow Mesenchymal Stem Cells with Overexpressed Nrf2 Inhibit Cardiac Fibrosis in Rats with Atrial Fibrillation. Cardiovasc Ther, 2022,2022:2687807
Yao L, Ye Y, Mao H, et al. MicroRNA-124 regulates the expression of MEKK3 in the inflammatory pathogenesis of Parkinson’s disease. J Neuroinflammation, 2018,15(1):13
Hu J, Shan Z, Hu K, et al. miRNA-223 inhibits epithelial-mesenchymal transition in gastric carcinoma cells via Sp1. Int J Oncol, 2016,49(1):325–335
Jaca A, Govender P, Locketz M, et al. The role of miRNA-21 and epithelial mesenchymal transition (EMT) process in colorectal cancer. J Clin Pathol, 2017,70(4):331–356
Domingues C, Serambeque BP, Laranjo CM, et al. Epithelial-mesenchymal transition and microRNAs: Challenges and future perspectives in oral cancer. Head Neck, 2018,40(10):2304–2313.
Lin F, Wu X, Zhang H, et al. A microrna screen to identify regulators of peritoneal fibrosis in a rat model of peritoneal dialysis. BMC Nephrol, 2015,16:48
Li X, Liu H, Sun L, et al. MicroRNA-302c modulates peritoneal dialysis-associated fibrosis by targeting connective tissue growth factor. J Cell Mol Med, 2019,23(4):2372–2383
Szeto CC, Chow KM, Kwan BC, et al. Peritoneal dialysis effluent miR-21 and miR-589 levels correlate with longitudinal change in peritoneal transport characteristics. Clin Chim Acta, 2017,464:106–112
Xi Y, Shen Y, Wu D, et al. CircBCAR3 accelerates esophageal cancer tumorigenesis and metastasis via sponging miR-27a-3p. Mol Cancer, 2022,21(1):145
Guo D, Li Y, Chen Y, et al. DANCR promotes HCC progression and regulates EMT by sponging miR-27a-3p via ROCK1/LIMK1/COFILIN1 pathway. Cell Prolif, 2019,52(4):e12628
Bello A K, Okpechi I G, Osman M A, et al. Epidemiology of peritoneal dialysis outcomes. Nat Rev Nephrol, 2022,18(12):779–793
Gu C, Feng J, Waqas A, et al. Technological Advances of 3D Scaffold-Based Stem Cell/Exosome Therapy in Tissues and Organs. Front Cell Dev Biol, 2021,9:709204
Fang Y, Garnier D, Lee TH, et al. PML-RARa modulates the vascular signature of extracellular vesicles released by acute promyelocytic leukemia cells. Angiogenesis, 2016,19(1):25–38
Vanherle S, Haidar M, Irobi J, et al. Extracellular vesicle-associated lipids in central nervous system disorders. Adv Drug Deliv Rev, 2020,159:322–331
Sabaratnam R, Geertsen L, Skjodt K, et al. In human nephrectomy specimens, the kidney level of tubular transport proteins does not correlate with their abundance in urinary extracellular vesicles. Am J Physiol Renal Physiol, 2019,317(3):F560–F571
Krediet R T. Ultrafiltration Failure Is a Reflection of Peritoneal Alterations in Patients Treated With Peritoneal Dialysis. Front Physiol, 2018,9:1815
Yanai K, Ishii H, Aomatsu A, et al. MicroRNAs in peritoneal fibrosis: a systematic review. Discov Med, 2018,26(145):271–280
PubMed Google Scholar
Shin HS, Ko J, Kim DA, et al. Metformin ameliorates the Phenotype Transition of Peritoneal Mesothelial Cells and Peritoneal Fibrosis via a modulation of Oxidative Stress. Sci Rep, 2017,7(1):5690
Ji S, Deng H, Jin W, et al. Beta-catenin participates in dialysate-induced peritoneal fibrosis via enhanced peritoneal cell epithelial-to-mesenchymal transition. FEBS Open Bio, 2017,7(2):265–273
Sung SA, Kim DH, Oh KH, et al. The Role of Cathepsin B in Peritoneal Fibrosis due to Peritoneal Dialysis. Int J Nephrol, 2019,2019:4150656
Yang A H, Chen JY, Lin JK. Myofibroblastic conversion of mesothelial cells. Kidney Int, 2003,63(4):1530–1539
Kalluri R, Weinberg RA. The basics of epithelial-mesenchymal transition. J Clin Invest, 2009,119(6):1420–1428
Zhao JL, Guo MZ, Zhu JJ, et al. Curcumin suppresses epithelial-to-mesenchymal transition of peritoneal mesothelial cells (HMrSV5) through regulation of transforming growth factor-activated kinase 1 (TAK1). Cell Mol Biol Lett, 2019,24:32
Haywood ME, Cocciolo A, Porter KF, et al. Transcriptome signature of ventricular arrhythmia in dilated cardiomyopathy reveals increased fibrosis and activated TP53. J Mol Cell Cardiol, 2020,139:124–134
Download references
Author information
Both authors contributed equally to this study.
Authors and Affiliations
Department of Nephrology, Shanghai University of Medicine & Health Sciences Affiliated Zhoupu Hospital, Shanghai, 201318, China
Jun-li Zhao, Qiu-nan Zhan, Miao Liu, Ting Zhang & Wen-wen Chu
Orthopedic Department, Guangming Traditional Chinese Medicine Hospital of Pudong New Area, Shanghai, 201399, China
You can also search for this author in PubMed Google Scholar
Corresponding author
Correspondence to Jun-li Zhao .

Ethics declarations
The authors declare that there is no conflict of interest with any financial organization or corporation or individual that can inappropriately influence this work.
Additional information
This study was supported by the Technology Development Program of Shanghai Pudong New District (No. PKJ2021-Y34) and the Excellent Young Medical Talent Training Program of Pudong Health Commission of Shanghai (No. PWRq2022-18).
Rights and permissions
Reprints and permissions
About this article
Zhao, Jl., Zhao, L., Zhan, Qn. et al. BMSC-derived Exosomes Ameliorate Peritoneal Dialysis-associated Peritoneal Fibrosis via the Mir-27a-3p/TP53 Pathway. CURR MED SCI (2024). https://doi.org/10.1007/s11596-024-2853-7
Download citation
Received : 09 July 2023
Accepted : 19 February 2024
Published : 16 April 2024
DOI : https://doi.org/10.1007/s11596-024-2853-7
Share this article
Anyone you share the following link with will be able to read this content:
Sorry, a shareable link is not currently available for this article.
Provided by the Springer Nature SharedIt content-sharing initiative
- peritoneal fibrosis
- bone marrow mesenchymal stem cell-derived exosomes
- epithelial-mesenchymal transition
- Find a journal
- Publish with us
- Track your research

Maintenance work is planned for Wednesday 1st May 2024 from 9:00am to 11:00am (BST).
During this time, the performance of our website may be affected - searches may run slowly and some pages may be temporarily unavailable. If this happens, please try refreshing your web browser or try waiting two to three minutes before trying again.
We apologise for any inconvenience this might cause and thank you for your patience.
Journal of Materials Chemistry B
Structure-activity relationships of aniline-based squaraines for distinguishable staining and bright two-photon fluorescence bioimaging in plant cells.
Organelle-selective vision provides insights into the physiological response of plants and crops to environmental stresses in sustainable agriculture ecosystems. Biological applications often require two-photon excited fluorophores with low phototoxicity, high brightness, deep penetration, and tuneable cell entry. We obtained three aniline-based squaraines (SQs) tuned from hydrophobic to hydrophilic characteristics by modifying terminal pendant groups and substituents, investigating their steady-state absorption and far-red-emitting fluorescence properties. The SQs exhibited two-photon absorption (2PA) ranging from 750 to 870 nm within the first biological spectral window; their structure-property relationships, corresponding to the 2PA cross sections (δ2PA), and structure differences were demonstrated. The maximum δ2PA value was ~1220 GM at 800 nm for hydrophilic SQ3. Distinct biological staining efficiency and selective SQ bioimaging were evaluated utilising the onion epidermal cell model. Contrary to the hydrophobic SQ1 results in the onion epidermal cell wall, amphiphilic SQ2 tagged the vacuole and nucleus; SQ3 tagged the vacuole. Distinguishable staining profiles in the roots and leaves were achieved. We believe this study is the first to demonstrate distinct visualisation efficiency induced by the structure differences of two-photon excited SQs. Our results can help establish the versatile roles of novel near-infrared-emitting SQs toward biological applications.
- This article is part of the themed collection: Journal of Materials Chemistry B HOT Papers
Supplementary files
- Supplementary information PDF (4522K)
Article information
Download citation, permissions.

N. Zhang, H. Chang, R. Miao, T. Liu, L. Ding and Y. Fang, J. Mater. Chem. B , 2024, Accepted Manuscript , DOI: 10.1039/D4TB00400K
To request permission to reproduce material from this article, please go to the Copyright Clearance Center request page .
If you are an author contributing to an RSC publication, you do not need to request permission provided correct acknowledgement is given.
If you are the author of this article, you do not need to request permission to reproduce figures and diagrams provided correct acknowledgement is given. If you want to reproduce the whole article in a third-party publication (excluding your thesis/dissertation for which permission is not required) please go to the Copyright Clearance Center request page .
Read more about how to correctly acknowledge RSC content .
Social activity
Search articles by author.
This article has not yet been cited.
Advertisements
Thank you for visiting nature.com. You are using a browser version with limited support for CSS. To obtain the best experience, we recommend you use a more up to date browser (or turn off compatibility mode in Internet Explorer). In the meantime, to ensure continued support, we are displaying the site without styles and JavaScript.
- View all journals
- My Account Login
- Explore content
- About the journal
- Publish with us
- Sign up for alerts
- Open access
- Published: 10 April 2024
FOXO1 is a master regulator of memory programming in CAR T cells
- Alexander E. Doan 1 na1 ,
- Katherine P. Mueller ORCID: orcid.org/0000-0002-1873-3638 2 , 3 , 4 na1 ,
- Andy Y. Chen ORCID: orcid.org/0000-0002-5018-355X 5 , 6 , 7 na1 ,
- Geoffrey T. Rouin 2 , 3 , 4 ,
- Yingshi Chen ORCID: orcid.org/0000-0002-5391-5103 2 , 3 , 4 ,
- Bence Daniel ORCID: orcid.org/0000-0002-2410-8767 5 , 7 , 8 , 9 nAff18 ,
- John Lattin 1 ,
- Martina Markovska 2 , 3 , 4 ,
- Brett Mozarsky 2 , 3 , 4 ,
- Jose Arias-Umana ORCID: orcid.org/0000-0002-7154-4780 2 , 3 , 4 ,
- Robert Hapke 2 , 3 , 4 ,
- In-Young Jung 10 ,
- Alice Wang ORCID: orcid.org/0000-0002-1789-8561 2 ,
- Peng Xu 1 ,
- Dorota Klysz ORCID: orcid.org/0000-0002-6733-6110 1 ,
- Gabrielle Zuern 2 , 3 , 4 ,
- Malek Bashti ORCID: orcid.org/0000-0003-0398-3367 1 ,
- Patrick J. Quinn ORCID: orcid.org/0000-0002-4406-727X 1 ,
- Zhuang Miao 9 ,
- Katalin Sandor ORCID: orcid.org/0000-0003-2892-4865 5 , 7 ,
- Wenxi Zhang 5 , 7 ,
- Gregory M. Chen 4 , 11 ,
- Faith Ryu 2 , 3 , 4 ,
- Meghan Logun ORCID: orcid.org/0000-0002-7274-811X 4 , 12 ,
- Junior Hall 2 , 13 , 14 ,
- Kai Tan 2 , 13 , 14 ,
- Stephan A. Grupp 2 , 13 , 14 ,
- Susan E. McClory 2 , 13 ,
- Caleb A. Lareau ORCID: orcid.org/0000-0003-4179-4807 5 , 7 , 15 ,
- Joseph A. Fraietta ORCID: orcid.org/0000-0001-7900-8993 4 , 10 , 11 , 14 ,
- Elena Sotillo ORCID: orcid.org/0000-0002-3993-1932 1 ,
- Ansuman T. Satpathy ORCID: orcid.org/0000-0002-5167-537X 5 , 7 , 15 ,
- Crystal L. Mackall ORCID: orcid.org/0000-0001-6323-4304 1 , 15 , 16 , 17 na2 &
- Evan W. Weber ORCID: orcid.org/0000-0001-5039-2899 2 , 3 , 4 , 13 , 14 , 15 na2
Nature ( 2024 ) Cite this article
23k Accesses
1 Citations
294 Altmetric
Metrics details
- Cancer immunotherapy
- Immunotherapy
A major limitation of chimeric antigen receptor (CAR) T cell therapies is the poor persistence of these cells in vivo 1 . The expression of memory-associated genes in CAR T cells is linked to their long-term persistence in patients and clinical efficacy 2 , 3 , 4 , 5 , 6 , suggesting that memory programs may underpin durable CAR T cell function. Here we show that the transcription factor FOXO1 is responsible for promoting memory and restraining exhaustion in human CAR T cells. Pharmacological inhibition or gene editing of endogenous FOXO1 diminished the expression of memory-associated genes, promoted an exhaustion-like phenotype and impaired the antitumour activity of CAR T cells. Overexpression of FOXO1 induced a gene-expression program consistent with T cell memory and increased chromatin accessibility at FOXO1-binding motifs. CAR T cells that overexpressed FOXO1 retained their function, memory potential and metabolic fitness in settings of chronic stimulation, and exhibited enhanced persistence and tumour control in vivo. By contrast, overexpression of TCF1 (encoded by TCF7 ) did not enforce canonical memory programs or enhance the potency of CAR T cells. Notably, FOXO1 activity correlated with positive clinical outcomes of patients treated with CAR T cells or tumour-infiltrating lymphocytes, underscoring the clinical relevance of FOXO1 in cancer immunotherapy. Our results show that overexpressing FOXO1 can increase the antitumour activity of human CAR T cells, and highlight memory reprogramming as a broadly applicable approach for optimizing therapeutic T cell states.
Similar content being viewed by others
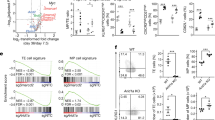
cBAF complex components and MYC cooperate early in CD8+ T cell fate
Ao Guo, Hongling Huang, … Douglas R. Green
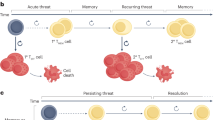
Stem-like exhausted and memory CD8+ T cells in cancer
Thomas Gebhardt, Simone L. Park & Ian A. Parish
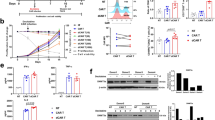
Low-dose decitabine priming endows CAR T cells with enhanced and persistent antitumour potential via epigenetic reprogramming
Yao Wang, Chuan Tong, … Weidong Han
More than 50% of patients who respond to CAR T cell therapies eventually relapse, and CAR T cells that target solid tumours have been largely ineffective 1 . The expression of memory T cell genes in patient CAR T cells is associated with durable persistence and disease control 2 , 3 , 4 , 5 , 6 , but the transcription factors that drive CAR T memory programs have not been identified. We previously showed 7 that providing rest to exhausted CAR T cells through transiently inhibiting CAR signalling promoted a memory-like phenotype and increased chromatin accessibility at motifs bound by the memory transcription factors TCF1 and FOXO1, raising the prospect that these transcription factors mediate memory programming in CAR T cells. Consistent with this notion, expression of TCF7 (which encodes TCF1) broadly correlates with responses to CAR T cell 2 , 5 , tumour-infiltrating lymphocyte (TIL) 8 and checkpoint blockade 9 , 10 therapies. In addition, FOXO1 directly regulates the expression of TCF7 and other canonical memory genes 11 , 12 and promotes the formation of central memory T cells in mice 12 , 13 , 14 .
Several groups have shown that pharmacological inhibition of AKT, a negative regulator of FOXO1, confers an early memory phenotype in human CAR T cells and TILs 15 , 16 , 17 , suggesting that FOXO1 also promotes memory in human T cells. To test the hypothesis that FOXO1 is required for memory programming and antitumour function in human CAR T cells, we performed phenotypic and functional experiments using CD19.28ζ or CD19.BBζ CAR T cells cultured in the presence of a selective FOXO1 small-molecule inhibitor 18 (FOXO1 i ) (Extended Data Fig. 1 ). FOXO1 i reduced the expansion and viability of CAR T cells, the frequency of CD8 + cells and the expression of memory-associated markers (CD62L, IL-7Rα and TCF1) in a dose-dependent manner, and concomitantly upregulated markers of short-lived effector or exhausted T cells (CD39, TIM-3 and TOX) (Extended Data Fig. 1b–e ).
We corroborated these data by using CRISPR–Cas9 to knock out FOXO1 (FOXO1 KO ) (Fig. 1a and Extended Data Fig. 2a ). FOXO1 KO CAR T cells showed a similar reduction in expansion and CD8 + frequency, diminished memory-associated markers and increased exhaustion-associated markers as compared with AAVS1 -edited control CAR T cells (Fig. 1b,c , Extended Data Fig. 2b–f and Supplementary Fig. 1 ). Because FOXO1 KO cells exhibited uniformly low CD62L surface expression, we used CD62L as a surrogate marker for FOXO1 editing by magnetically purifying CD62L lo FOXO1 KO cells for bulk RNA sequencing (RNA-seq) (Extended Data Fig. 2g,h ). FOXO1 KO cells upregulated activation- and exhaustion-associated genes ( TOX , NR4A1 , FOS and CD69 ), downregulated memory and FOXO1 target genes ( IL7R and CCR7 ) and exhibited less naive-like and more exhausted gene-expression signatures (Fig. 1d,e and Extended Data Fig. 2i ).
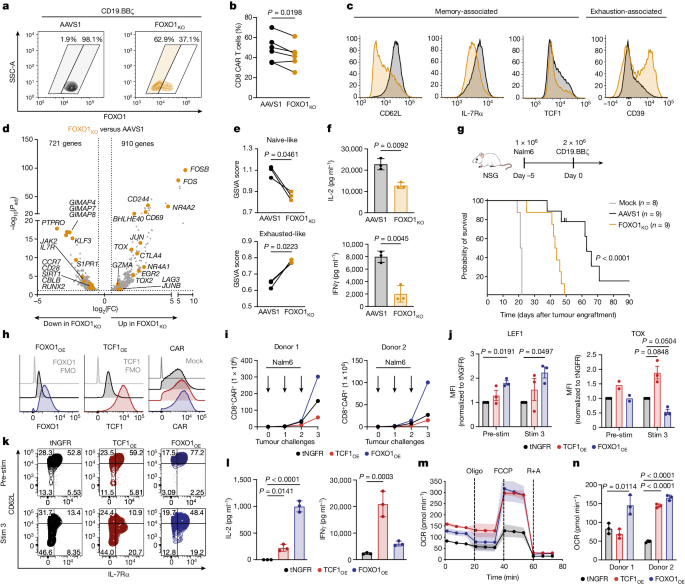
a – g , CRISPR–Cas9 gene editing of AAVS1 (AAVS1) or FOXO1 (FOXO1 KO ) in CD19.BBζ CAR T cells. a – c , Flow cytometric analysis of FOXO1 knockout efficiency ( a ), percentage of CAR + CD8 + cells at day 14 ( b ) and memory- and exhaustion-associated markers in CAR + CD8 + cells ( c ). Shaded areas in a represent gates used in phenotypic analyses. One representative donor is shown in a and c ( n = 6 donors). d , Volcano plot of DEGs in CD62L lo FOXO1 KO versus AAVS1 (Bonferroni-adjusted P < 0.05 with absolute log 2 -transformed fold change (abs(log 2 (FC)) > 0.5). e , GSVA using T cell gene signatures 55 . f , Cytokine secretion in response to Nalm6 leukaemia cells from one representative donor ( n = 4 donors). g , Stress test Nalm6 xenograft model. Top, schematic. Bottom, survival curves of Nalm6-engrafted mice treated with mock T cells or gene-edited CD19.BBζ cells. Data show two donors tested in two independent experiments ( n = 8 or 9 mice per group). Data in d and e include n = 3 donors. h – n , CAR T cells overexpressing truncated NGFR (tNGFR), TCF1-P2A-tNGFR (TCF1 OE ) or FOXO1-P2A-tNGFR (FOXO1 OE ). h , Flow cytometric analysis of FOXO1, TCF1 and CD19.28ζ expression from one representative donor ( n = 8 donors). FMO, fluorescence minus one. i – k , Serial restimulation of CD19.BBζ cells with Nalm6. CD8 + CAR T cell expansion ( i ) and flow cytometric analysis of memory- and exhaustion-associated markers ( j , k ). j , Mean ± s.e.m. of normalized mean fluorescence intensity (MFI) ( n = 2 or 3 donors). k , One representative donor ( n = 4 donors). l , HA.28ζ cytokine secretion (day 13) in response to 143B osteosarcoma cells from one representative donor ( n = 4 donors). m , n , HA.28ζ seahorse analysis (day 13) ( n = 2 donors). m , Oxygen consumption rate (OCR) (mean ± s.d. of 11 technical replicates from one representative donor). Oligo, oligomycin; R+A, rotenone and antimycin. n , Spare respiratory capacity. Data in f , l , n are mean ± s.d. of three technical replicates. Statistical comparisons were performed using paired two-tailed Student’s t -test ( b , e ), two-sided Welch’s t -test ( f ), log-rank Mantel–Cox test ( g ) and repeated-measures one-way ANOVA with Geisser–Greenhouse correction ( j ) or one-way ANOVA with Dunnett’s test ( l , n ) .
Source Data
FOXO1 i and FOXO1 KO cells also exhibited attenuated killing and/or cytokine secretion after tumour challenge (Fig 1f and Extended Data Fig. 1f,g ), consistent with a model in which FOXO1 restrains exhaustion and/or terminal differentiation in human T cells, similar to reports in mice 14 , 19 , 20 , 21 , 22 . We corroborated these results using an in vitro CAR T cell exhaustion model (HA.28ζ CAR), in which antigen-independent tonic CAR signalling induces features of exhaustion within approximately one week 7 , 23 . Knockout of FOXO1 in HA.28ζ cells accelerated the manifestation of exhaustion markers and dysfunction (Extended Data Fig. 2j,k ). We next modelled chronic antigen stimulation in vivo by infusing a sub-therapeutic dose of CD19.BBζ cells into leukaemia-bearing mice 7 , 24 . Knockout of FOXO1 significantly reduced CAR T cell tumour control and survival (Fig. 1g ). These observations show that endogenous FOXO1 promotes memory and is required for optimal antitumour function of CAR T cells.
FOXO1 overexpression preserves a memory phenotype
Among the genes induced by FOXO1 is TCF7 , which has been broadly implicated in memory programming, stemness and antitumor activity in human and mouse T cells 2 , 5 , 8 , 10 , 25 , 26 , 27 , 28 , 29 , 30 , 31 , 32 , 33 . Thus, we sought to determine whether the overexpression of FOXO1 and/or TCF1 could enhance the function of human CAR T cells. Human T cells were co-transduced with a retrovirus expressing a CAR and a second virus expressing truncated NGFR (tNGFR) as a control or a bicistronic vector containing tNGFR and either TCF1 (TCF1 OE ) or FOXO1 (FOXO1 OE ) (Extended Data Fig. 3a ). This approach enabled high levels of transcription factor overexpression and equivalent CAR expression across conditions (Fig. 1h ). Notably, CD19.BBζ cells expressing FOXO1 OE , but not TCF1 OE , exhibited increased baseline expression of memory-associated surface markers and transcription factors, including endogenous TCF1 (refs. 12 , 13 ) (Extended Data Fig. 3b,c ).
TCF1 OE and FOXO1 OE cells that were serially rechallenged with Nalm6 leukaemia both exhibited enhanced cytokine secretion compared with controls (Extended Data Fig. 3d ), but only FOXO1 OE increased CD8 proliferation and memory marker expression while suppressing the levels of TOX (Fig. 1i–k and Extended Data Fig. 3e ). By contrast, TCF1 OE increased the expression of TOX and CD39 relative to tNGFR controls, consistent with a more exhausted or effector-like phenotype (Fig. 1j and Extended Data Fig. 3e ). We corroborated these results in cells expressing the tonic signalling HA.28ζ CAR, in which both TCF1 OE and FOXO1 OE cells showed enhanced function, but only FOXO1 OE promoted a memory-like surface phenotype (Fig. 1l and Extended Data Fig. 3f–h ).
Because the metabolism of memory T cells favours oxidative phosphorylation (OXPHOS) relative to glycolysis, we used Seahorse to assess whether transcription factor overexpression induces memory-like metabolic profiles. FOXO1 OE and TCF1 OE showed increased OXPHOS and superior metabolic fitness compared with tNGFR controls. The degree of FOXO1 OE -mediated metabolic reprogramming was more marked in exhausted HA.28ζ cells (Fig. 1m,n ) compared with those expressing CD19.28ζ (Extended Data Fig. 3i,j ), consistent with the notion that FOXO1 OE counteracts the exhaustion program.
FOXO1 OE promotes a memory-like gene signature
We hypothesized that FOXO1 and TCF1 induce disparate gene-expression programs because overexpression of each endowed CAR T cells with distinct cell-surface phenotypes and functionality (Fig. 1 ). Therefore, we performed bulk RNA-seq on purified CD4 + or CD8 + FOXO1 OE and TCF1 OE T cells expressing HA.28ζ to model settings of chronic antigen stimulation. Principal component analysis (PCA) showed that FOXO1 OE and TCF1 OE CAR T cells clustered separately from tNGFR and had a greater number of unique differentially expressed genes (DEGs) than shared genes (Fig. 2a,b and Extended Data Fig. 4a–c ). PCA also showed that transcription factor overexpression was a stronger driver of differential gene expression than CD4 + or CD8 + cell identity (Extended Data Fig. 4b ), confirming that FOXO1 OE and TCF1 OE promote divergent gene-expression programs in both subsets.
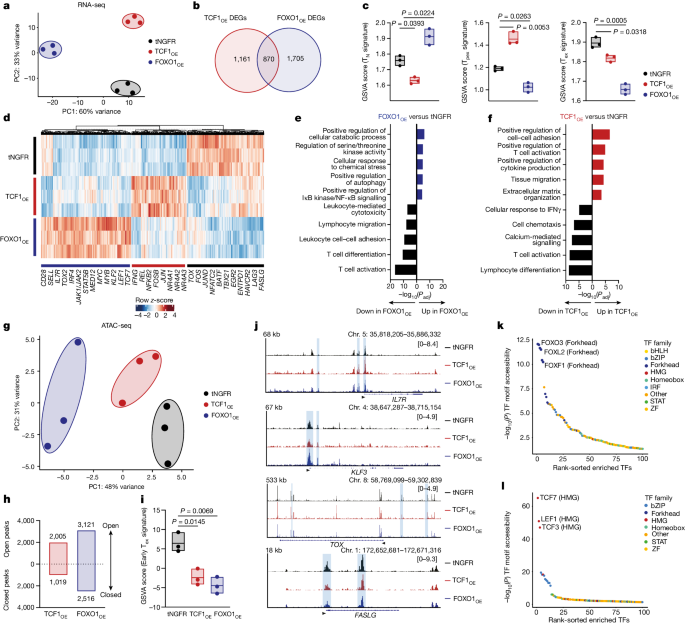
a – l , Bulk RNA-seq ( a – f ) and ATAC-seq ( g – l ) in tNGFR + CD8 + HA.28ζ CAR T cells ( n = 3 donors). a , RNA-seq PCA. b , Venn diagram showing unique and shared DEGs in TCF1 OE and FOXO1 OE compared with tNGFR (Bonferroni-adjusted P < 0.05 with abs(log 2 (FC) > 0.5). c , GSVA of DEGs using naive (T N ), T pex and exhausted (T ex ) T cell signatures 55 . Centre line represents mean score. d , Heat map and hierarchical clustering of DEGs. Genes of interest are highlighted. The colour bar shows normalized z -scores for each DEG. e , f , GO term analyses showing curated lists of the top upregulated and downregulated processes in FOXO1 OE ( e ) and TCF1 OE ( f ) versus tNGFR (Benjamini–Hochberg-adjusted P ). g , ATAC-seq PCA. h , Number of differentially accessible peaks compared with tNGFR ( P < 0.05 with abs(log 2 FC) > 0.5). i , GSVA of differentially accessible peaks using an early T ex cell epigenetic signature 9 . Centre line represents mean score. j , Chromatin accessibility tracks for the IL7R , KLF3 , TOX and FASLG loci, for one representative donor. k , l , Rank-ordered plots of differentially accessible transcription factor (TF)-binding motifs in FOXO1 OE ( k ) and TCF1 OE ( l ) versus tNGFR. ZF, zinc-finger. Statistical comparisons were performed using DESeq2 ( b , d , h , k , l ), one-sided hypergeometric test ( e , f ) and repeated-measures one-way ANOVA with Dunnett’s test ( c , i ).
Gene set variation analysis (GSVA) showed that FOXO1 OE promoted a naive-like and less terminally exhausted gene signature (Fig. 2c ). Consistent with these data, HA.28ζ FOXO1 OE cells upregulated genes associated with memory ( SELL , IL7R , LEF1 and TCF7 ) and downregulated those associated with exhaustion ( TOX , HAVCR2 , ENTPD1 and CD244 ) (Fig. 2d and Extended Data Fig. 4d,e ). Despite the fact that previous literature has implicated FOXO1 in regulatory T (T reg ) cell biology 34 , 35 , FOXO1 OE did not enforce a T reg gene signature (Extended Data Fig. 4f ). Gene ontology (GO) and ingenuity pathway analysis (IPA) showed that FOXO1 OE promoted autophagy, cellular catabolism and naive-associated transcription factor gene-expression networks ( TCF7 and LEF1 ) and diminished effector transcription factor networks ( ID2 , PRDM1 and TBX21 ) (Fig. 2e,f and Extended Data Fig. 4g,h ). By contrast, TCF1 OE cells exhibited high expression of exhaustion-associated transcription factors of the NR4A family, a progenitor exhausted T (T pex ) cell-like gene signature (Fig. 2c ), and were enriched in effector gene-expression pathways (for example, cell–cell adhesion, T cell activation and cytokine production) (Fig. 2d,f and Extended Data Fig. 4d,e,g ). Similar results were obtained in CD19.28ζ cells (Extended Data Fig. 4i–k ); however, FOXO1 OE resulted in a greater number of DEGs in tonic signalling HA.28ζ CAR T cells compared with those expressing CD19.28ζ, indicating more marked transcriptional reprogramming by FOXO1 during chronic stimulation.
TCF1 and FOXO1 are considered pioneer factors owing to their ability to directly bind to condensed chromatin and recruit chromatin remodelling machinery 36 , 37 . To test whether TCF1 OE and/or FOXO1 OE induce chromatin remodelling, we performed a bulk assay for transposase-accessible chromatin with sequencing (ATAC-seq) in TCF1 OE and FOXO1 OE CAR T cells (Supplementary Fig. 2 ). PCA confirmed that both transcription factors promoted global changes to chromatin accessibility compared with tNGFR controls (Fig. 2g and Extended Data Fig. 5a ). This effect was most evident in tonically signalling HA.28ζ cells, in which FOXO1 OE clustered separately from tNGFR and TCF1 groups and showed more differentially accessible peaks (around 5,600; P < 0.05) compared with TCF1 OE cells (around 3,000) (Fig. 2g,h ). Most of the differentially accessible peaks in FOXO1 OE were open, consistent with the ability of FOXO1 ability to perturb core histone–DNA contacts 37 .
HA.28ζ FOXO1 OE cells showed increased accessibility at FOXO1 target gene loci ( IL7R and KLF3 ), reduced accessibility at exhaustion-associated loci ( TOX and FASLG ) and a decreased exhaustion-like epigenetic signature compared with tNGFR cells (Fig. 2i,j ), consistent with transcriptomic data. Of note, DNA-binding motifs for transcription factors of the forkhead box and HMG-box families were the top-ranked differentially accessible motifs in FOXO1 OE and TCF1 OE cells, respectively (Fig. 2k,l and Extended Data Fig. 5b,c ), supporting a model in which overexpressed FOXO1 and TCF1 induce local chromatin remodelling. Paradoxically, FOXO1 OE cells also showed increased accessibility at transcription factor motifs associated with effector function (for example, b-ZIP and NF-κB p65) (Extended Data Fig. 5d,e ).
These data show that FOXO1 OE induces memory and naive-like gene-expression programs during chronic stimulation, whereas TCF1 OE promotes a T pex -like program, consistent with the role identified for TCF1 in chronic infection and cancer 25 , 26 , 38 , 39 . In addition, FOXO1 OE induces a unique epigenetic state that supports effector function while maintaining memory programming.
FOXO1 OE enhances CAR T function against leukaemia
Because FOXO1 OE was effective at promoting memory (Fig. 1 ), we hypothesized that further increasing the activity of FOXO1 might endow CAR T cells with a more stable memory phenotype. We generated a humanized version of a nuclear-restricted variant of FOXO1 (FOXO1 3A ), which is insensitive to AKT-mediated nuclear export 19 (Extended Data Fig. 6a–c and Supplementary Fig. 3 ). FOXO1 3A increased the surface expression of FOXO1 target genes to a similar extent to FOXO1 OE (Extended Data Fig. 6d,e ). However, FOXO1 3A expression induced a divergent transcriptomic profile that was de-enriched in T cell activation genes and led to blunted in vitro cytokine secretion and cytotoxicity compared with FOXO1 OE (Extended Data Fig. 6f–i ). These observations raised the prospect that excessive nuclear FOXO1 activity might promote a stable memory phenotype and oppose effector function 21 .
To assess function in a protracted model in which memory programming might be important for sustained antitumor activity, we used a stress test xenograft model in which leukaemia-bearing mice received a sub-therapeutic dose of CD19.28ζ (Fig. 3a ) or CD19.BBζ (Extended Data Fig. 7a ) CAR T cells. FOXO1 OE markedly enhanced the tumour control of CAR T cells compared with tNGFR, whereas TCF1 OE showed no benefit (Fig. 3a and Extended Data Fig. 7a,b ). Similar results were obtained in a curative Nalm6 model, in which FOXO1 OE cells exhibited increased expansion and persistence compared with TCF1 OE and tNGFR cells (Fig. 3b and Extended Data Fig. 7c–e ). FOXO1 3A provided a modest survival advantage compared with tNGFR, but FOXO1 3A cells exhibited delayed expansion and reduced levels of tumour control compared with FOXO1 OE cells (Fig. 3a–c ), consistent with the notion that FOXO1 3A partially opposes effector function. To assess the recall response to secondary antigen challenge—a hallmark feature of memory T cells 40 —we rechallenged nearly cured mice with a high dose of Nalm6 (Fig. 3b,c and Extended Data Fig. 7c–e ). Only FOXO1 OE cells re-expanded after rechallenge and conferred a survival advantage, showing that FOXO1 OE endows CAR T cells with superior in vivo effector- and memory-like functions compared with tNGFR, TCF1 OE or FOXO1 3A .
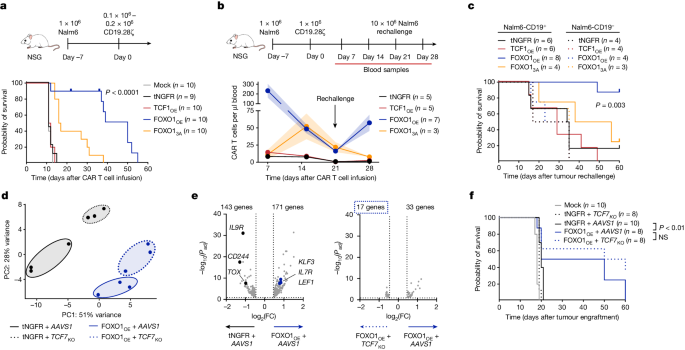
a , Subcurative doses of 0.1 × 10 6 –0.2 × 10 6 tNGFR + CD19.28ζ cells were infused into Nalm6-bearing mice seven days after engraftment. Schematic (top) and survival curve (bottom) are shown; n = 9-10 mice per group. b – d , Curative doses of 1 × 10 6 tNGFR + CD19.28ζ cells were infused into Nalm6-bearing mice seven days after engraftment. Mice were rechallenged with 10 × 10 6 CD19 + or CD19 − Nalm6 on day 21 after CAR T cell infusion ( n = 2 donors tested in 2 independent experiments). b , Rechallenge Nalm6 model. Schematic (top) and quantification (bottom) of circulating human CD45 + CAR T cells. Mean ± s.e.m. of n = 3–7 mice per group from one representative donor. c , Survival curve after rechallenge ( n = 3–8 mice per group pooled from 2 donors). d – f , CD19.28ζ cells overexpressing tNGFR or FOXO1 OE were gene-edited to knock out AAVS1 (control; AAVS1 ) or TCF7 ( TCF7 KO ). d , RNA-seq PCA. e , Volcano plots of DEGs; n = 3 donors (Bonferroni-adjusted P < 0.05 with abs(logFC) > 0.5). f , Stress test Nalm6 model. tNGFR + CD19.28ζ cells (0.6 × 10 6 cells) were infused into Nalm6-bearing mice seven days after engraftment. Survival curve is shown ( n = 8–10 mice per group). a , c , f show pooled data from two donors tested in two independent experiments. Statistical comparisons were performed using log-rank Mantel–Cox test ( a , c , f ) and DESeq2 ( e ). NS, not significant.
TCF7 is not required for FOXO1 OE reprogramming
To investigate the mechanism by which FOXO1 OE reprograms CAR T cells and increases in vivo antitumour activity, we generated a variant of FOXO1 with lower-affinity DNA binding (FOXO1 DBD ) 41 . FOXO1 DBD showed a modest reduction in DNA binding, and its expression in CAR T cells perturbed FOXO1-mediated transcriptional and epigenetic reprogramming (Extended Data Fig. 8a–d ). Mice that received CD19.28ζ FOXO1 DBD cells showed reduced survival in a Nalm6 leukaemia stress test model compared to those that were infused with FOXO1 OE cells (Extended Data Fig. 8e ), indicating that FOXO1 OE DNA binding is crucial for augmented antitumour activity.
The FOXO1 target gene, TCF7 ), is highly upregulated in FOXO1 OE cells (Extended Data Figs. 3c and 4d ). Although TCF1 OE did not increase the potency of CAR T cells, we reasoned that high endogenous levels of TCF7 and expression kinetics in FOXO1 OE could be mechanistically important for FOXO1 OE reprogramming. Notably, knockout of TCF7 in the context of FOXO1 OE had negligible effects on FOXO1 OE transcriptional reprogramming and in vivo antitumour activity (Fig. 3d–f and Extended Data Fig. 8f ). Thus, FOXO1 OE reprogramming requires DNA binding but not transcription of the memory-associated transcription factor and target gene, TCF7 .
FOXO1 OE enhances CAR T function in solid tumours
To determine whether FOXO1 was also capable of increasing the activity of CAR T cells against solid tumours, we infused tNGFR or FOXO1 OE HER2.BBζ CAR T cells into 143B osteosarcoma-bearing NSG mice. Consistent with leukaemia models, FOXO1 OE cells showed durable antitumour activity and persistence (Fig. 4a–e and Extended Data Fig. 9a–d ). Tumour-infiltrating FOXO1 OE cells exhibited transcriptomic reprogramming, were enriched in gene signatures associated with T cell killing, effector function and tissue residence, and showed negligible differences in human T reg signatures 42 , 43 (Fig. 4f–h and Extended Data Fig. 9e–i ). Of note, intratumoral FOXO1 OE cells did not have a canonical memory-like phenotype but were enriched in a FOXO1 OE transcriptomic signature derived from bulk RNA-seq studies (Fig. 4h ), suggesting that exogenous FOXO1 remains active in the tumour microenvironment.
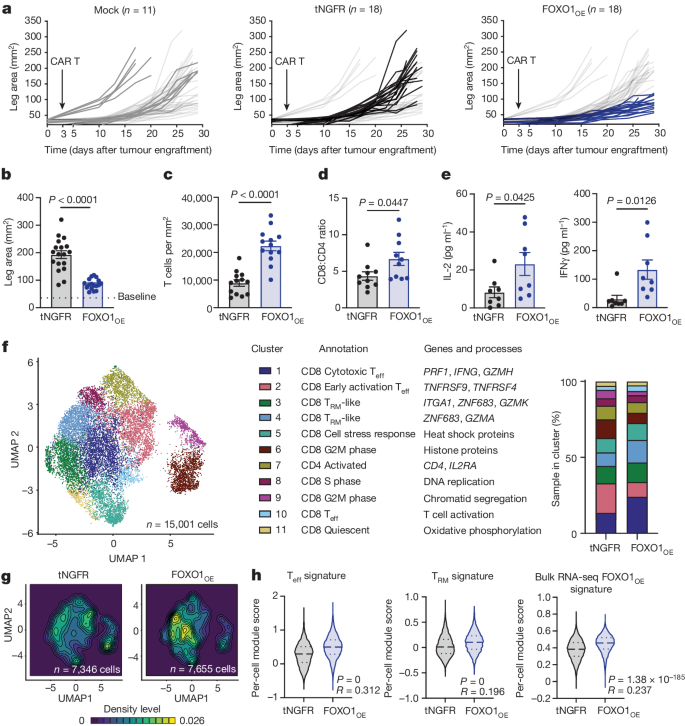
A total of 5 × 10 6 mock or tNGFR + Her2.BBζ CAR T cells expressing tNGFR or FOXO1 OE were infused into 143B-bearing mice three days after engraftment. a , b , Tumour measurements over time ( a ) and on day 25–29 ( b ). One FOXO1 OE mouse has been omitted in b owing to tumour-independent death before day 25. Data were pooled from three donors tested in three independent experiments ( n = 11–18 mice per group). c – e , Analysis of day-29 CAR TILs. c , Total CAR TILs ( n = 13 mice per group). d , Ratio of CD8 + to CD4 + CAR TILs. One representative donor ( n = 10 mice per group). e , CAR TIL IL-2 and IFNγ secretion after ex vivo stimulation with 143B ( n = 13 mice per group). Data in c – e were pooled from two donors tested in two independent experiments. f – h , Single-cell RNA-seq on day-29 CAR TILs. Cells were sorted and pooled from n = 5 mice per group from one donor. f , Left, uniform manifold approximation and projection (UMAP) of CAR TILs. Eleven clusters were identified with k -nearest neighbours clustering, and were annotated manually (middle). Right, sample distribution by cluster. T eff , T effector cell; T RM , tissue resident memory T cell. g , Sample distribution within the UMAP. h , T eff , T RM and FOXO1 OE -associated transcriptional signatures. Long dashed lines represent the mean and short dashed lines represent the top and bottom quartiles. Data in b – e are mean ± s.e.m. Statistical comparisons were performed using two-tailed Student’s t -test ( b – e ) and two-sided Wilcoxon rank-sum test ( h ).
Together, these data show that FOXO1 OE increases the in vivo expansion, persistence and tumour control of CAR T cells in a TCF7 -independent manner, whereas TCF1 OE provides no measurable benefit. FOXO1 OE -mediated enhancements are dependent on DNA binding and nuclear export, which suggests that tuning or signal regulation mediated by nuclear shuttling is important for effective FOXO1-mediated memory programming.
FOXO1 activity correlates with response to T cell therapies
FOXO1 target genes, including TCF7 , were enriched in pre-infusion CAR T cells that mediate clinical responses in patients 2 , 5 (Extended Data Fig. 10a,b ), raising the possibility that endogenous FOXO1 activity might predict potent antitumour activity in clinical CAR T products. Paradoxically, however, FOXO1 transcript levels in pre-infusion CD19.BBζ cells were not associated with response to therapy or survival in adults with chronic lymphocytic leukaemia (CLL) (Fig. 5a and Extended Data Fig. 10c ). Because FOXO1 is regulated mainly post-translationally rather than transcriptionally 15 , we hypothesized that the activity of FOXO1 could be better approximated by the aggregate expression of FOXO1 target genes. We therefore identified a FOXO1 ‘regulon’ consisting of 41 overlapping DEGs that were downregulated in FOXO1 KO cells and upregulated in FOXO1 OE cells (Fig. 5b ). The FOXO1 regulon included putative FOXO1 target genes (for example, SELL and KLF3 ), but was made up largely of genes that have not previously been associated with memory programming (Supplementary Table 1 ). In contrast to FOXO1 transcript, the FOXO1 regulon was significantly enriched in pre-infusion CAR T cells from patients with CLL who exhibited complete or partial responses with transformed disease, and was associated with in vivo CAR T cell expansion and overall survival (Fig. 5c,d and Extended Data Fig. 10d ). TCF7 did not reach statistical significance in FOXO1 KO experiments and was therefore not included in the FOXO1 regulon; however, regulon score significantly correlated with the TCF7 transcript in patient CAR T cells, suggesting that the regulon is an accurate readout for FOXO1 transcriptional activity (Fig. 5e ).
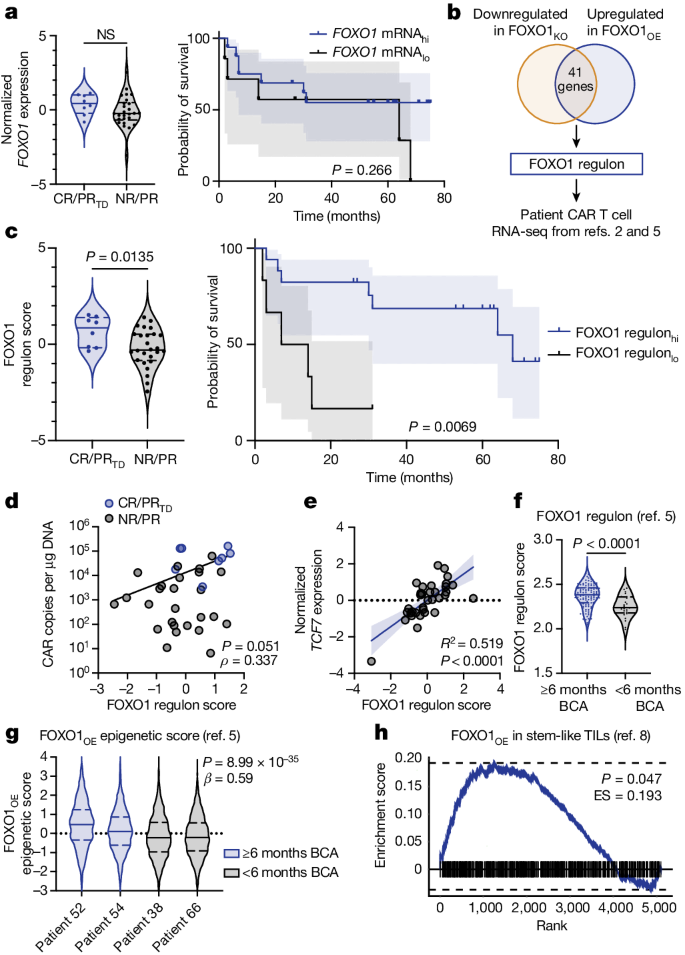
a – e , Single-sample gene set enrichment analysis (ssGSEA) on RNA-seq from pre-infusion, CAR-stimulated CTL019 cells from patients with CLL 2 (complete responder (CR), n = 5; partial responder with transformed disease (PR TD ), n = 3; partial responder (PR), n = 5; non-responder (NR), n = 21). a , FOXO1 ssGSEA for patient outcomes (left) and overall survival (right). b , The FOXO1 regulon was generated using FOXO1 KO and FOXO1 OE bulk RNA-seq data and then applied to published datasets 2 , 5 ; n = 3 donors. c , FOXO1 regulon ssGSEA (data from ref. 2 ) for patient outcomes (left) and overall survival (right). d , Least squares regression (dark line) of FOXO1 regulon score and peak CAR T cell expansion. e , Simple linear regression (dark line) of TCF7 expression and FOXO1 regulon score. Dark lines in a , c represent patient survival curves and shaded areas in a , c , e represent 95% confidence intervals. Dots in d , e represent individual samples (blue, CR/PR TD ; grey, NR/PR). f , FOXO1 regulon ssGSEA for pre-manufactured effector T cells from paediatric patients with B-ALL with durable (six or more months of B cell aplasia (BCA); n = 33 patients) or short (less than six months of BCA; n = 27 patients) CAR T cell persistence 5 . g , An epigenetic signature derived from FOXO1 OE ATAC-seq was applied to pre-manufactured T cell single-cell ATAC-seq data from paediatric patients 5 . Data show FOXO1 OE epigenetic signature scores for patients with durable (patient 52, n = 616 cells; patient 54, n = 2,959 cells) and short (patient 38, n = 2,093 cells; patient 66, n = 2,355 cells) CAR T cell persistence. h , GSEA using FOXO1 OE DEGs and DEGs derived from CD39 − CD69 − TILs from adult patients with melanoma 8 . ES, enrichment score. Violin plots in a , c , f , g show minima and maxima; solid lines represent the mean and long dashed lines represent the top and bottom quartiles. Statistical comparisons were performed using two-tailed Mann–Whitney test ( a , left; c , left; f ), log-rank Mantel–Cox test ( a , right; c , right), Spearman correlation ( d , e ), two-sided Wald test ( g ) and two-sided Kolmogorov–Smirnov test ( h ).
The FOXO1 regulon was also enriched in pre-manufactured effector T cells from children with B cell acute lymphoblastic leukaemia (B-ALL) who exhibited durable CAR T cell persistence 5 (Fig. 5f ), supporting the notion that FOXO1 activity broadly correlates with the efficacy of CAR T cells. Because both FOXO1 and TCF1 mediate chromatin remodelling 36 , 37 , 44 , 45 , 46 (Fig. 2 ), we next used epigenetic signatures derived from our ATAC-seq analyses to interrogate single-cell ATAC-seq data from paediatric CAR T cells 5 . Consistent with FOXO1 regulon transcriptomic data, the FOXO1 OE epigenetic signature was significantly enriched in patient T cells that were associated with durable persistence, whereas the TCF1 OE signature was not (Fig. 5g and Extended Data Fig. 10e ). Finally, FOXO1 OE DEGs were enriched in stem-like CD39 − CD69 − TILs that were highly predictive of the response to TIL therapy in adult patients with melanoma 8 , whereas TCF1 OE DEGs were de-enriched (Fig. 5h and Extended Data Fig. 10f ).
In this study, we tested the hypothesis that overexpressing memory-associated transcription factors could reprogram CAR T cells to durably persist and maintain antitumor activity. We focused our efforts on FOXO1, on the basis of studies that have implicated this transcription factor in memory programming 12 , 13 , 14 , 19 , 20 , 21 , 46 , 47 , 48 , 49 , 50 , 51 and our previous work in which we showed that exhaustion reversal and memory programming were associated with enhanced chromatin accessibility at FOXO1-binding motifs 7 . FOXO1 overexpression induced memory gene-expression programs and chromatin remodelling, mitigated exhaustion and substantially improved persistence and antitumour function in four distinct xenograft models. Its effect was independent of CAR binder, co-stimulatory domain and tumour type, highlighting the broad applicability of this pro-memory program across CAR T cell products.
There is a vast body of literature describing the role of FOXO1 in promoting T cell memory and persistence in mice 12 , 13 , 14 , 19 , 20 , 21 , 46 , 47 , 48 , 49 , 50 , 51 ; however, FOXO1 biology in human T cells remains poorly understood. Because the activity of FOXO1 is regulated at the post-translational level rather than through changes in transcription and is therefore hidden in RNA-seq data, the role of FOXO1 in cancer immunology and immunotherapy is likely to have been considerably underappreciated. Our study is the first, to our knowledge, to show that endogenous FOXO1 is required for memory gene expression and optimal antitumour function in engineered human T cells, which is consistent with the effects of Foxo1 knockout in mouse models of acute and chronic infection 14 , 19 , 20 . We further show that endogenous FOXO1 restrains exhaustion in human T cells, because deleting FOXO1 induced an exhaustion-like phenotype and CAR T cell dysfunction.
Notably, FOXO1 activity in pre-infusion CAR T cells and TILs strongly correlated with clinical responses, underscoring the importance of FOXO1 in T-cell-based cancer immunotherapies. Paradoxically, expression of a nuclear-restricted variant (FOXO1 3A ) altered FOXO1 reprogramming and attenuated the antitumour function of CAR T cells, supporting the notion that optimal FOXO1 activity involves intermittent and/or context-dependent regulation. Indeed, others have shown that transient expression of FOXO1 3A can induce partial memory reprogramming in human CAR T cells without impairing effector function 15 , 52 , 53 . Further work is needed to determine how FOXO1 expression levels and kinetics affect the function of CAR T cells and whether FOXO1 is relevant in other therapeutic modalities, such as immune checkpoint blockade.
We also interrogated TCF1, a transcription factor that defines stem-like or memory T cell populations that exhibit an increased capacity to respond to immune checkpoint blockade 2 , 5 , 8 , 10 , 25 , 26 , 27 , 28 , 29 , 30 , 31 , 32 , 33 . Of note, overexpressing TCF1 did not enforce memory gene-expression programs or enhance antitumour activity in vivo, which contradicts reports in mice 27 , 28 . Instead, TCF1 OE cells exhibited a gene-expression signature associated with T pex cells, and manifested functional hallmarks of exhaustion during chronic stimulation, consistent with other studies 39 , 54 . Thus, our results raise the possibility that constitutive TCF1 overexpression skews human engineered T cells towards a more exhausted or T pex cell-like state, and/or that TCF7 -expressing T pex cells do not have a substantial role in CAR T cell responses.
An alternative interpretation posits that FOXO1, rather than TCF1, is mainly responsible for endowing tumour-reactive T cells with a stem-like or progenitor phenotype, and that TCF7 expression is merely a readout for FOXO1 activity. Indeed, deletion of endogenous TCF7 in FOXO1 OE did not affect FOXO1-mediated transcriptional reprogramming or augmented antitumour function in vivo. Surface markers and transcription factors that are often co-expressed in TCF7 + cells are FOXO1 target genes 29 , and our empiric FOXO1 regulon significantly correlated with TCF7 expression and clinical responses in samples of CAR T cells from patients, further supporting this notion. Conditional deletion of Foxo1 in mature mouse T cells diminished the frequency of Tcf7 -expressing T pex cells 14 , suggesting that FOXO1 might promote cell states that are normally associated with high levels of Tcf7 expression. Future mechanistic studies are warranted to determine the precise roles of FOXO1 and TCF1 in human engineered and non-engineered T cells during cancer immunotherapy.
In summary, we show that FOXO1-driven transcriptional and epigenetic programs are associated with engineered and non-engineered T cells that expand, persist and promote clinical responses in patients with cancer. Overexpression of FOXO1 increases the activity of CAR T cells through memory reprogramming, and TCF1 is insufficient to induce CAR T cell memory and persistence. Our results suggest that FOXO1 represents a major therapeutic axis that can be exploited to improve the efficacy of T-cell-based cancer immunotherapies.
Primary human T cells
For experiments completed at Stanford, buffy coats from anonymous, consenting healthy donors were obtained from the Stanford University Blood Center under an University Institutional Review Board-exempt protocol or obtained from a human peripheral blood leukopak (STEMCELL Technologies). CD3 + cells were isolated using the RosetteSep Human T Cell Enrichment Kit, Lymphoprep density gradient medium and SepMate-50 tubes according to the manufacturer’s protocol (STEMCELL Technologies). For experiments completed at the Children’s Hospital of Philadelphia (CHOP), purified CD3 + healthy donor T cells were obtained from the University of Pennsylvania Human Immunology Core. All purified T cells were cryopreserved in CryoStor CS10 medium (STEMCELL Technologies).
Cell lines were obtained from ATCC and stably transduced to express markers as follows: 143B osteosarcoma cells express GFP and firefly luciferase with or without CD19, Nalm6 B-ALL cells express GFP and firefly luciferase with or without GD2. Single-cell clones were chosen for high antigen expression. The 143B and Nalm6 cells were cultured in Dulbecco’s modified Eagle’s medium (DMEM) and RPMI 1640, respectively, and both were supplemented with 10% fetal bovine serum (FBS), 10 mM HEPES and 1× penicillin–streptomycin–glutamate (Gibco). Nalm6 and 143B cell lines and engineered versions of these cell lines were previously authenticated via STR fingerprinting prior to their use in this study. HEK293 cells were originally obtained from the National Cancer Institute. Cells were frequently tested for mycoplasma using the Lonza MycoAlert Mycoplasma Detection kit.
Design of CAR and transcription factor constructs
The CAR constructs used in this study include CD19.28ζ, CD19.BBζ, anti-GD2 HA.28ζ and Her2.BBζ. Codon-optimized TCF1, FOXO1 or FOXO1 3A sequences and a P2A ribosomal skip sequence were generated as Gene Blocks by IDT and constructed in MSGV retroviral vectors. The tNGFR-only construct does not contain a P2A ribosomal skip sequence. The FOXO1 DBD construct was generated by two-step mutagenic NEBuilder HiFi DNA Assembly (New England BioLabs). All plasmids were amplified by transformation into Stellar Competent Escherichia coli (Takara Bio), and sequences were validated by sequencing (Elim Biopharmaceuticals).
Retrovirus production
To generate retrovirus, ten million 293GP cells were plated on a 15-cm BioCoat poly- d -lysine cell culture plate (Corning) and fed with 20 ml of DMEM supplemented with 10% FBS, 10 mM HEPES and 1× penicillin–streptomycin–glutamate (Gibco) 24 h before transfection. Transfection was performed by mixing a room-temperature solution of 3.4 ml Opti-MEM (Gibco) + 135 μl Lipofectamine 2000 (Invitrogen) (solution 1) with a second solution of 3.4 ml Opti-MEM + 11 μg RD114 packaging plasmid DNA + 22 μg MSGV retroviral plasmid of interest (solution 2) by slow dropwise addition of solution 2 to solution 1. The combined solution 1 and 2 mixture was incubated for 30 min at room temperature, after which the medium was replaced on 293GP cells, and 6.5 ml of the combined solution was added to the plates in a slow, dropwise manner. The next day, the culture medium was replaced on 293GP cells. At 48 h after transfection, the viral supernatant was collected from the cells and the culture medium was replaced; supernatant collection was repeated at 72 h. At each step, the supernatant was spun down to remove cells and debris, and frozen at −80 °C for future use.
T cell activation and culture
T cells were thawed in warm water after removal from liquid nitrogen and then washed with T cell medium (AIM-V (Gibco) supplemented with 5% FBS, 10 mM HEPES, 1× penicillin–streptomycin–glutamate and 100 U ml −1 recombinant human IL-2 (Peprotech) or RPMI (Gibco) supplemented with 10% FBS, 10 mM HEPES, 1× penicillin–streptomycin–glutamate and 100 U ml −1 recombinant human IL-2). Human T-Expander αCD3/CD28 Dynabeads (Gibco) were washed and added to T cells at a volume of 30 μl resuspended beads per million T cells. T cells and beads were then resuspended at a concentration of 500,000 T cells per ml in T cell medium (day 0 for all assays). Forty-eight and 72 hours after activation, T cells were transduced (see ‘Retroviral transduction’). Ninety-six hours after activation, beads were removed by magnetic separation using a DynaMag column (Invitrogen). T cells were fed with fresh T cell medium every 48–72 h and were maintained at a density of 0.5 ×10 6 cells per ml after feeding. For FOXO1 i experiments, T cells were provided with fresh complete T cell medium and vehicle control (dimethyl sulfoxide; DMSO) or AS1842856 (EMD Millipore) every 2–3 days from days 4 to 15 after activation.
Retroviral transduction
T cells were transduced with retrovirus on days 2 and 3 after activation for all experiments. In brief, 12- or 24-well, non-tissue-culture-treated plates were coated with 1 ml or 500 μl, respectively, of 25 μg ml −1 Retronectin (Takara) in PBS and placed at 4 °C overnight. The next day, plates were washed with PBS then blocked with 2% bovine serum albumin (BSA) + PBS for 10 min. Retroviral supernatants were added and plates were centrifuged at 32 °C for 2 h at 2,500 g . Viral supernatants were subsequently removed and T cells were added to each virus-coated well at a density of 1 × 10 6 T cells per well for 12-well plates and 0.5 × 10 6 T cells per well for 24-well plates.
Cell selection
tNGFR isolations were performed using either Miltenyi MACS sorting or STEMCELL EasySep sorting unless otherwise stated. For Miltenyi MACS sorting, cells were resuspended in FACS buffer and stained with biotin anti-human CD271 (tNGFR) antibody (BioLegend). Cells were washed with PBS, 0.5% BSA and 2 mM EDTA (MACS buffer), resuspended in MACS buffer and mixed with Streptavidin MicroBeads (Miltenyi), then washed again with MACS buffer and passed through an LS Column for positive selection inside a MACS separator (Miltenyi). For STEMCELL EasySep sorting, cells were isolated using the manufacturer’s protocol for the EasySep Human CD271 Positive Selection Kit II (STEMCELL Technologies) with an EasyEights EasySep Magnet (STEMCELL Technologies). After isolation, cells were immediately mixed with warm complete T cell medium, counted and resuspended at 500,000 per ml.
For RNA-seq experiments on FOXO1 KO cells, CD62L lo CAR + cells were isolated by negative selection, first by staining cells with anti-CD62L-PE and then by following the EasySep PE Positive Selection Kit II protocol according to the manufacturer’s instructions (STEMCELL Technologies). For RNA-seq and ATAC-seq experiments on tNGFR, TCF1 OE and FOXO1 OE cells, CD8 + tNGFR + CAR T cells were isolated before sequencing using the EasySep Human CD8+ T Cell Isolation Kit (STEMCELL Technologies). For in vivo analysis of tumour-infiltrating CAR T cells, CD45 + T cells were isolated from tumours using the EasySep Release Human CD45 Positive Selection Kit (STEMCELL Technologies) according to the manufacturer’s instructions.
CRISPR–Cas9 gene editing
To interrogate the role of endogenous FOXO1 in CAR T cell function, CRISPR–Cas9 was used to delete a sequence directly upstream of the FOXO1 DNA-binding domain. On day 4 after activation, retrovirally transduced CAR T cells were removed from activation beads by magnetic separation. Twenty-microlitre reactions were prepared by resuspending one million CAR T cells in P3 buffer immediately before electroporation with the P3 Primary Cell 4D Nucleofector Kit (Lonza). Ribonucleoproteins were prepared by complexing 0.15 ng of sgRNA targeting FOXO1 or AAVS1 (Synthego) with 5 µg Alt-R S.p. Cas9 Nuclease (IDT, 1081058) before adding the cell suspension to each reaction. For AAVS1 edits, a previously validated sgRNA sequence (5′-GGGGCCACUAGGGACAGGAU-3′) was used. For FOXO1 , two separate sgRNAs were used in tandem, at equal concentrations (5′-UUGCGCGGCUGCCCCGCGAG-3′ and 5′-GAGCUUGCUGGAGGAGAGCG-3′). For TCF7 gene editing, we used a previously validated sgRNA 56 (5′-UCAGGGAGUAGAAGCCAGAG-3′) for bulk RNA-seq experiments performed at CHOP. A separate sgRNA (5′-UUUUCCAGGCCUGAAGGCCC-3′) was designed and validated at Stanford, and used for in vivo experiments. The reaction was pulsed with the EH115 program on a Lonza 4D Nucleofector. Cells were recovered immediately in 260 µl of warm complete AIM-V medium supplemented with 500 U ml −1 IL-2 in round-bottom 96-well plates and expanded into 1 ml fresh medium within 24 h. Cells were maintained at 0.5 × 10 6 cells per ml to 1.0 × 10 6 cells per ml in well plates until day 14–16 for functional and phenotypic characterization. On days 14–16, knockout efficiency was determined by intracellular transcription factor staining (Cell Signaling, 58223) followed by flow cytometry.
Flow cytometry
CAR T cells were washed twice in FACS buffer (PBS + 2% FBS) and stained with fluorophore-conjugated surface antibodies for 30 min on ice. Cells were washed twice with FACS buffer before analysis. Intracellular stains were performed with the same initial surface stain, after which cells were fixed, permeabilized and stained using the FoxP3 Transcription Factor Staining Buffer Set according to the manufacturer’s protocol (eBioscience). Anti-human FOXO1 (clone C29H4) and anti-human TCF1 (C36D9) antibodies were purchased from Cell Signaling. The 1A7 anti-14G2a idiotype antibody used to detect the HA CAR was obtained from the NCI and conjugated using the Dylight 650 antibody labelling kit (Thermo Fisher Scientific). The anti-FMC63 idiotype antibody was manufactured by GenScript and fluorescently conjugated using the Dylight 650 antibody labelling kit. Cell-surface antibodies were used at a 1:100 dilution during staining, with the exception of anti-14g2a and anti-FMC63, which were used at a 1:1,000 dilution. Intracellular antibodies were used at a 1:50 dilution and live/dead staining was used at a 1:1,000 dilution. Cells were analysed with either a BD Fortessa running FACS Diva software, or a Cytek Aurora using SpectroFlo v.3.1.0. Downstream analyses were performed using Cytek SpectroFlo v.3.1.0 and FlowJo v.10.8.1 Software. All reagents are listed in Supplementary Table 2 . A representative gating strategy for FOXO1 KO and FOXO1 OE experiments is shown in Supplementary Fig. 1 . In experiments in which we stained for Annexin V, cells were gated on all singlets, excluding debris but not excluding dead or dying T cells. For MFI quantification, background subtraction was performed using either unstained or FMO samples. The MFI quantification in Extended Data Fig. 1e was not background subtracted owing to negative MFI values in some control samples.
Cytokine secretion assays
A total of 5 × 10 4 CAR T cells were co-cultured with 5 ×10 4 tumour cells in 200 μl of complete T cell medium (AIM-V or RPMI) without IL-2 in a 96-well plate, all in triplicate. Twenty-four hours after co-culture, culture supernatants were collected, diluted 20- to 100-fold and analysed for IL-2 and IFNγ using ELISA MAX kits (BioLegend) and Nunc Maxisorp 96-well ELISA plates (Thermo Fisher Scientific). Absorbance readings were collected on a Tecan Spark plate reader or a BioTek Synergy H1 running Gen5 v.2.00.18. For FOXO1 i assays, the co-culture medium included concentrations of AS1842856 that were used during T cell expansion.
IncuCyte killing assay
A total of 5 × 10 4 GFP + tumour cells and T cells corresponding to a 1:1, 1:2, 1:4, 1:8 and/or 1:16 effector:target ratios were co-cultured in 300 μl of T cell medium without IL-2 in 96-well flat-bottom plates. Plates were imaged at 10× zoom with 4–9 images per well every 2–4 h for 96 h using the IncuCyte ZOOM S3 Live-Cell analysis system (Essen BioScience/Sartorius). The total integrated GFP intensity per well or total GFP area (μm 2 per well) were used to analyse the expansion or contraction of Nalm6 or 143B cells, respectively. All GFP intensity and area values were normalized to the first imaging time point ( t = 0). For FOXO1 i assays, the co-culture medium included concentrations of AS1842856 that were used during T cell expansion.
Repeat stimulation assay
CAR T cells were activated and transduced, and tNGFR + cells were isolated as described above. Cells were cultured in AIM-V with IL-2 until day-14 ‘pre-stim’ assays, including flow cytometry, cytokine secretion and IncuCyte as described above. On day 14, co-cultures were set up comprising 5 × 10 T cells and 2 × 10 6 Nalm6 tumour cells suspended in AIM-V without IL-2 at a final concentration of 5 × 10 5 total cells per ml. Co-cultures were fed with 5 ml of AIM-V without IL-2 on day 3 of culture. On day 3 of the repeat stimulation co-culture, CAR T cells were again assayed by cytokine secretion, IncuCyte killing assay and flow cytometry as described above. This process was repeated for a total of four co-cultures such that the cytokine and IncuCyte assays were set up for four serial stimulations on days 14, 17, 20 and 23 on cells that had been stimulated with Nalm6 tumour zero, one, two and three previous times, respectively, for a total of four serial stimulations by the end of the experiment. Cells were analysed by flow cytometry on day 7 of co-culture, such that T cells were co-cultured with tumour on days 14, 17, 20 and 23 and analysed on days 21, 24, 27 and 30, respectively.
Seahorse assay
Metabolic analyses were performed using Seahorse Bioscience Analyzer XFe96. In brief, 0.2 × 10 6 cells were resuspended in extracellular flux assay medium supplemented with 11 mM glucose, 2 mM glutamine and 1 mM sodium pyruvate, and plated on a Cell-Tak (Corning)-coated microplate allowing the adhesion of CAR T cells. Mitochondrial activity and glycolytic parameters were measured by the oxygen consumption rate (OCR) (pmol min −1 ) and extracellular acidification rate (ECAR) (mpH min −1 ), respectively, with the use of real-time injections of oligomycin (1.5 M), carbonyl cyanide ptrifluoromethoxyphenylhydrazone (FCCP; 0.5 M) and rotenone and antimycin (both at 0.5 M). Respiratory parameters were calculated according to the manufacturer’s instructions (Seahorse Bioscience). Reagent sources are listed in Supplementary Table 2 .
Immunoblotting
Chromatin-bound and soluble proteins were separated as previously described 23 . In brief, cytoskeletal (CSK) buffer was prepared using 100 mM NaCl, 300 mM sucrose, 3 mM MgCl 2 , 10 mM PIPES (pH 6.8), 0.1% IGEPAL CA-630, 4 µg ml −1 aprotinin, 10 µg ml −1 leupeptin, 4 µg ml −1 pepstatin and 2 mM PMSF. After washing with ice-cold PBS, cell pellets were lysed with CSK buffer for 20 min on ice. Samples were centrifuged at 1,500 g for 5 min and the soluble fraction was separated and cleared by centrifugation at 15,870 g for 10 min. The protein concentration of the soluble fraction was determined by DC protein assay (Bio-Rad, 5000116). The remaining pellet containing the chromatin-bound fraction was washed twice with CSK buffer, centrifuging at 1,500 g for 5 min. Chromatin-bound proteins were resuspended in CSK buffer and 1× Pierce Reducing Sample Buffer (Thermo Fisher Scientific, 39000) and boiled for 5 min for solubilization. The soluble fraction was supplemented with Pierce Reducing Sample Buffer to achieve 1× and boiled for 5 min. For immunoblotting, equal amounts of soluble and chromatin-bound fraction for each sample were analysed by SDS–polyacrylamide gel electrophoresis and transferred to nitrocellulose membranes (Bio-Rad, 1704158). Membranes were blocked for 30 min in 5% milk in TBST (1× Tris-buffered saline containing 0.1% Tween-20). After washing with TBST, membranes were incubated with anti-FOXO1 antibody (1:1,000; Cell Signaling, 2880, clone C29H4) overnight at 4 °C. Next, membranes were washed with TBST and incubated with anti-mouse (1:10,000, Cell Signaling, 7074) or anti-rabbit (1:10,000, Cell Signaling, 7076) IgG conjugated to horseradish peroxidase for 1 h at room temperature. Membranes were visualized using Clarity Western ECL Substrate (Bio-Rad, 1705060) and the ChemiDoc Imaging System and Image Lab Touch Software v.3.0 (Bio-Rad). After visualization, membranes were stripped using a mild stripping buffer (1.5% glycine, 0.1% SDS, 1% Tween-20, pH 2.2). The previous steps were repeated for detection of soluble (1:5,000 GAPDH; Cell Signaling, 97166, clone D4C6R) and chromatin-bound (1:1,000 Lamin A; Cell Signaling, 86846, clone 133A2) fraction loading controls. Densitometry analyses were performed using Fiji v.2.14.0/1.5 f.
Mouse xenograft models
NOD/SCID/ Il2rg −/ − (NSG) mice were bred, housed and treated under Stanford University APLAC- or CHOP ACUP-approved protocols. Six-to-eight-week-old mice were healthy, immunocompromised, drug- and test-naive and unused in other procedures. Mice were housed at the Stanford Veterinary Service Center (VSC) or CHOP Department of Veterinary Services (DVR) in a barrier facility with a 12-h light–dark cycle, and mice were kept at a temperature of 20–23 °C (CHOP) or 20–26 °C (Stanford) with humidity ranging from 30–70%. Five mice were housed in each cage in aerated racks with ample bedding, food and water. For mice that became sick, solid feeds were switched to liquid feeds to facilitate eating. Mice were monitored daily by trained VSC and DVR staff under the supervision of a veterinarian who reported excess morbidity immediately and/or euthanized mice for humane reasons. Mice were euthanized if end-point criteria were met, which included 143B tumour sizes exceeding 1.2 cm or Nalm6 bioluminescence greater than 5 × 10 11 photons per second, or if evidence of extensive disease occurred (for example, inability to ambulate, groom or eat, cachexia, excessive loss of fur, hunched posture or other signs of disability); whichever came first. Tumour injection sites were chosen so as not to interfere with the mouse’s normal body functions, such as ambulation, eating, drinking, defecation and/or urination. In Nalm6-bearing mice, 2 × 10 5 to 1 × 10 7 cells in 100–200 μl of sterile PBS were engrafted by tail vein injection (TVI). In 143B osteosarcoma models, 1 × 10 6 to 3 × 10 6 cells in 100 μl sterile PBS were engrafted by intramuscular injection into the flank. Mice were randomized prior to CAR T cell infusion to ensure equal tumour burden across groups. CAR T cells were engrafted by TVI at doses and schedules noted in the main text. Nalm6 engraftment, expansion and clearance were measured by intraperitoneal injection of luciferin and subsequent imaging by a Spectrum IVIS bioluminescence imager and quantified using Living Image software v.4.7.3 (Perkin Elmer), or by a Lago X imager and quantified using Aura software v.4.0.7 (Spectral Instruments Imaging), all under isoflurane anaesthesia. The 143B tumour size was monitored by caliper measurements. Tumor and T cell injections were performed by technicians who were blinded to treatments and expected outcomes.
Mouse tissue analyses
Peripheral blood was sampled from live, isoflurane-anaesthetized mice by retro-orbital blood collection. Fifty microlitres of blood was labelled with surface antibodies, lysed using FACS Lysing Solution (BD) and quantified using CountBright Absolute Counting Beads (Thermo Fisher Scientific), then analysed on a BD Fortessa cytometer. For phenotypic analysis of spleen and tumours, mice were euthanized and tissues were mechanically dissociated and washed twice in PBS. Spleens were placed in a 6-cm Petri dish and filtered through a sterile 70-µm cell strainer. Tumours were mechanically and chemically dissociated with Collagenase IV and DNAse in HBSS and incubated at 37 °C with shaking for 30 min. Cells were mashed through a sterile 70-µm cell strainer before washing with PBS. Cells from both spleens and tumours were spun down at 450 g for 5 min at 4 °C, then treated with ACK lysis buffer for 3 min on ice. Cell suspensions were washed twice with PBS and CAR T cells were isolated by positive selection using the EasySep Release Human CD45 Positive Selection Kit. Cells were stained for markers of interest and analysed on a Cytek Aurora using SpectroFlo Software 3.1.0.
Bulk RNA-seq
A total of 0.5 × 10 6 –1 × 10 6 T cells were pelleted by centrifugation and flash-frozen. Pellets were thawed on ice and processed using either an RNEasy Plus Mini Kit or an AllPrep DNA/RNA Micro Kit (for simultaneous DNA and RNA isolation) (QIAGEN) according to the manufacturer’s instructions. Total RNA was quantified using either a Qubit Fluorometer or a DeNovix DS-11 FX Spectrophotometer/Fluorometer and sequenced using a 150 bp paired-end read length and around 50 million read pairs per sample (Novogene).
Bulk RNA-seq processing and analysis
We processed the sequencing data using the nf-core RNA-seq pipeline ( https://nf-co.re/rnaseq ). In brief, we performed quality control of the fastq files using FastQC and trimmed the filtered reads with Trim Galore software. The trimmed fastq files resulting from the experiment were aligned to the hg38 human genome using STAR. Salmon was then used to generate a gene-by-sample count matrix for downstream analysis. PCA was performed on read counts that were processed using the variance-stabilizing transformation, and plots were generated from the top 1,000 variable genes across samples. To correct for batch effects by donor, the removeBatchEffect function in the limma package was used. Differential analysis of gene expression was performed using the DESeq2 v.3.16 package, with an absolute log 2 -transformed fold change ≥0.5 and false discovery rate (FDR) < 0.05. To create a heat map, differential genes were aggregated, and expressions were standardized with z -scores across samples. The k -means clustering algorithm with Pearson correlation as the distance metric was used to cluster the genes. Pathway analysis of the differential genes and grouped genes in the heat map was performed using QIAGEN Ingenuity Pathway Analysis 2022 Winter Release and clusterProfiler v.4.6.2. Cell-type enrichment was performed through the single-sample extension of gene set enrichment analysis (ssGSEA) in the GSVA v.1.46.0 R package using signature genes from previous studies 8 , 55 using R v.4.1.0.
Single-cell RNA-seq library preparation and sequencing
To generate single-cell RNA-seq libraries of tumour-infiltrating CAR T cells, Her2 + tumours were collected from five mice per condition, and human CD45 + cells were isolated by NGFR selection as described above (see ‘Cell selection’). Tumour-infiltrating CAR T cells were further purified by sorting human CD3 + TILs from each isolate using a Cytek Aurora Cell Sorter. A total of 20,000 CAR TILs were sorted from each tumour and pooled across five mice per group. Cells were barcoded and sequencing libraries were generated using the 10X Chromium Next GEM Single Cell 3’ v.3.1 kit (10X Genomics) according to the manufacturer’s instructions. Libraries were sequenced at the CHOP High Throughput Sequencing Core on an Illumina NovaSeq 6000 with an average read depth of 50,000 reads per cell.
Single-cell RNA-seq processing and analysis
FASTQ files were generated and aligned to the genome with Cell Ranger v.7.1.0, using a custom GRCh38 reference genome containing the Her2.BBζ CAR sequence. Low-quality cells with fewer than 300 or more than 7,500 genes or more than 10% mitochondrial reads were removed using Seurat v.4.3.0 (ref. 57 ) in R. Doublets were identified using DoubletFinder v.2.0.3 and removed. Filtered samples were normalized using SCTransform before integration. The integrated dataset was scaled, and UMAP dimensionality reduction was performed using the top 30 principal components. Unsupervised Louvain clustering was performed on a shared nearest neighbour graph at a final resolution of 0.6. FindAllMarkers (Seurat) was used to identify DEGs in each cluster, and GO analyses were performed for each cluster using ClusterProfiler v.4.6.2. DEGs and GO processes were used to manually annotate each cluster, and contaminating CD3 − tumour cells were removed. Differential gene analyses between samples were performed using FindMarkers (Seurat) using the Wilcoxon rank-sum test with Bonferroni correction. Gene set scores for T eff , T RM and T reg cell subtypes were calculated with AddModuleScore (Seurat), using curated gene lists from a previous study 58 (Extended Data Fig. 9g–i ). AddModuleScore was also used to calculate a per-cell FOXO1 transcriptional activity score, using the top 100 upregulated genes in CD8 + HA.28ζ CAR T cells overexpressing FOXO1 versus tNGFR (Fig. 2 ). Gene set scores for T eff , T RM and FOXO1 signatures were generated for pan CD3 + T cells (Fig. 4i ; individual genes are shown in Extended Data Fig. 9g–i ). The T reg gene set score was computed for the CD4 + subset of cells expressing ≥1 CD4 mRNA counts and no detectable CD8A counts (Extended Data Fig. 9f ).
Bulk ATAC-seq processing
CD8 + tNGFR + CAR T cells were isolated using the EasySep Human CD8+ T Cell Isolation Kit. A total of 150,000 CD8 + T cells were slow-frozen in BamBanker (Bulldog Bio) cell preservation medium. Approximately 100,000 CAR T cells were washed in ice-cold PBS and subjected to nuclei isolation using the following lysis buffer: 10 mM Tris-HCl pH 7.5, 10 mM NaCl, 3 mM MgCl 2 , 0.1% Tween-20, 0.1% NP40, 0.01% Digitonin and 1% BSA. After washing the cells, 50 μl lysis buffer was added to each sample and cells were resuspended by pipetting. Nuclear pellets were centrifuged and resuspended in the transposase reaction containing 10.5 μl H 2 O, 12.5 μl 2× TD buffer and 2 μl Tn5 transposase in a total of 25 μl. The reaction was incubated for 30 min at 37 °C. The reaction was stopped by the addition of 75 μl TE buffer and 500 μl PB buffer (QIAGEN), followed by column purification per the manufacturer’s recommendation (QIAGEN, Minelute Kit). DNA was eluted from the columns in 22 μl H 2 O. PCR reactions were set up as follows: 21 μl DNA, 25 μl Phusion master mix (NEB) and 2 μl of each barcoded PCR primer (ApexBio, K1058). Fifteen PCR cycles were run for each sample. Reactions were cleaned up with AMPure XP beads according to the recommendations of the manufacturer. Libraries were quantified with a Qubit fluorometer and fragment analysis was performed with Bioanalyzer. Libraries were sequenced on a NovaSeq 6000 sequencer.
Bulk ATAC-seq analysis
ATAC-seq libraries were processed using the pepatac pipeline ( http://pepatac.databio.org/ ) with default options. In brief, fastq files were trimmed to remove adapter sequences, and then pre-aligned to the mitochondrial genome to exclude mitochondrial reads. To ensure the accuracy of downstream analysis, multimapping reads aligning to repetitive regions of the genome were filtered from the dataset. Bowtie2 was then used to align the reads to the hg38 genome. SAMtools was used to identify uniquely aligned reads, and Picard was used to remove duplicate reads. The resulting deduplicated and aligned BAM file was used for downstream analysis. Peaks in individual samples were identified using MACS2 and compiled into a non-overlapping 500-bp consensus peak set. In brief, the peaks were resized to 500 bp width and ranked by significance. The peaks that overlapped with the same region were selected by ranks and the most significant peak was retained. The peak-sample count matrix was generated using ChrAccR with the default parameters of the run_atac function. Signal tracks for individual samples were generated within the pepatac pipeline. These tracks were then merged by group using WiggleTools to produce a comprehensive view of the data across all samples.
On the basis of our analysis of the peak-sample count matrix, the DESeq2 v.3.16 package was used to identify differential peaks across different conditions, with a threshold of an absolute log 2 -transformed fold change greater than 0.5 and P value less than 0.05. Adjusted P values were not used owing to donor variability. To generate PCA plots, we first extracted a variance-stabilized count matrix using the vst function in DESeq2. Next, we corrected for batch effects by donor using the removeBatchEffect function in the limma library. Finally, we generated PCA plots using the corrected matrix with the plotPCA function using the top 2,000 most variable peaks. We aggregated differential peaks across conditions, standardized the peak signals using z -scores across samples and performed k -means clustering to generate a chromatin accessibility heat map. Motif enrichments of differential peaks and grouped peaks were searched with HOMER and findMotifsGenome.pl with default parameters. The enrichment of cell-type-specific regulatory elements were performed with the gchromVAR package. In brief, this method weights chromatin features by log 2 -transformed fold changes of cell-type-specific regulatory elements from a previous report 9 and computes the enrichment for each cell type versus an empirical background matched for GC content and feature intensity.
Identification and analysis of the FOXO1 regulon
The FOXO1 regulon gene set was generated by intersecting downregulated differential genes (log 2 -transformed fold change < −0.25, FDR < 0.05) in FOXO1 KO cells and upregulated differential genes (log 2 -transformed fold change > 0.5, FDR < 0.05) in FOXO1 OE cells (Supplementary Table 1 ). Regulon enrichment scores were calculated using ssGSEA in the GSVA R package on a previous RNA expression dataset 2 .
For regulon analyses of single-cell ATAC-seq data, the processed Signac data objects of CAR T products profiled by single-cell ATAC-seq were obtained from a previous study 5 . To account for sample-to-sample variability, the mean fragments in peaks per cell were downsampled for consistency between donors. Furthermore, donors PT48 and PT51 were excluded on the basis of low data quality after examination of quality control statistics, including per-library transcription start site enrichment. Using the epigenetic signature for FOXO1 and TCF1 overexpression (Fig. 2 ), we computed the per-cell epigenetic signature per factor using the chromVAR workflow as previously described for related T cell signatures derived from bulk experiments. To test for differences in responder/non-responder associations with this signature, we performed an ordinary least squares regression with the per-cell z -score against the donor’s BCA status at 6 months, adjusting for individual patient ID. Statistical significance was based on the Wald test statistic of the coefficient for the responder term in the two regressions for each factor.
For regulon analyses of the CLL CD19 CAR T cell clinical dataset, the gene-expression data table for activated CD19 CAR T cell products from patients with CLL was obtained from a previous report 2 . The enrichment of the FOXO1 signature was analysed using ssGSEA as previously described and performed using the R package GSVA v.1.46.0. To compare the ssGSEA enrichment scores between responders and non-responders, a Mann–Whitney test was conducted. To statistically determine optimal stratification points for survival analysis, we compared candidate stratification points on the basis of hazard ratio and P value as previously described. The survival analysis was conducted with a log-rank (Mantel–Cox) test using GraphPad Prism v.9.5.0.
Statistical analyses
Unless otherwise stated, statistical analyses for significant differences between groups were conducted using one- or two-way analysis of variance (ANOVA) with Bonferroni, Tukey’s or Dunnett’s multiple comparisons test, or with a Student’s or Welch’s t -test using GraphPad Prism v.9.4.1. In experiments in which same-donor samples were compared across two conditions, we performed a paired Student’s t -test. Survival curves were compared using the log-rank Mantel–Cox test. Statistical methods were not used to predetermine sample sizes.
Reporting summary
Further information on research design is available in the Nature Portfolio Reporting Summary linked to this article.
Data availability
Transcription factor constructs will be made available through material transfer agreements when possible. The bulk RNA-seq, ATAC-seq and single-cell RNA-seq datasets were aligned to human genome hg38; they have been deposited in the NCBI Gene Expression Omnibus and are accessible through the accession number GSE255416 . Source data are provided with this paper.
Code availability
All code associated with this paper have been deposited to the Weber Lab GitHub repository ( https://github.com/Weber-Lab-CHOP/FOXO1_2024 ) 59 .
Weber, E. W., Maus, M. V. & Mackall, C. L. The emerging landscape of immune cell therapies. Cell 181 , 46–62 (2020).
Article CAS PubMed PubMed Central Google Scholar
Fraietta, J. A. et al. Determinants of response and resistance to CD19 chimeric antigen receptor (CAR) T cell therapy of chronic lymphocytic leukemia. Nat. Med. 24 , 563–571 (2018).
Deng, Q. et al. Characteristics of anti-CD19 CAR T cell infusion products associated with efficacy and toxicity in patients with large B cell lymphomas. Nat. Med. 26 , 1878–1887 (2020).
Bai, Z. et al. Single-cell antigen-specific landscape of CAR T infusion product identifies determinants of CD19-positive relapse in patients with ALL. Sci. Adv. 8 , eabj2820 (2022).
Chen, G. M. et al. Integrative bulk and single-cell profiling of premanufacture T-cell populations reveals factors mediating long-term persistence of CAR T-cell therapy. Cancer Discov. 11 , 2186–2199 (2021).
Kirouac, D. C. et al. Deconvolution of clinical variance in CAR-T cell pharmacology and response. Nat. Biotechnol. 41 , 1606–1617 (2023).
Weber, E. W. et al. Transient rest restores functionality in exhausted CAR-T cells through epigenetic remodeling. Science 372 , eaba1786 (2021).
Krishna, S. et al. Stem-like CD8 T cells mediate response of adoptive cell immunotherapy against human cancer. Science 370 , 1328–1334 (2020).
Article ADS CAS PubMed PubMed Central Google Scholar
Satpathy, A. T. et al. Massively parallel single-cell chromatin landscapes of human immune cell development and intratumoral T cell exhaustion. Nat. Biotechnol. 37 , 925–936 (2019).
Sade-Feldman, M. et al. Defining T Cell states associated with response to checkpoint immunotherapy in melanoma. Cell 175 , 998–1013 (2018).
Fabre, S. et al. FOXO1 regulates L-selectin and a network of human T cell homing molecules downstream of phosphatidylinositol 3-kinase. J. Immunol. 181 , 2980–2989 (2008).
Article CAS PubMed Google Scholar
Kim, M. V., Ouyang, W., Liao, W., Zhang, M. Q. & Li, M. O. The transcription factor Foxo1 controls central-memory CD8 + T cell responses to infection. Immunity 39 , 286–297 (2013).
Hess Michelini, R., Doedens, A. L., Goldrath, A. W. & Hedrick, S. M. Differentiation of CD8 memory T cells depends on Foxo1. J. Exp. Med. 210 , 1189–1200 (2013).
Article PubMed Google Scholar
Utzschneider, D. T. et al. Active maintenance of T cell memory in acute and chronic viral infection depends on continuous expression of FOXO1. Cell Rep. 22 , 3454–3467 (2018).
Klebanoff, C. A. et al. Inhibition of AKT signaling uncouples T cell differentiation from expansion for receptor-engineered adoptive immunotherapy. JCI Insight 2 , e95103 (2017).
Article PubMed PubMed Central Google Scholar
Crompton, J. G. et al. Akt inhibition enhances expansion of potent tumor-specific lymphocytes with memory cell characteristics. Cancer Res. 75 , 296–305 (2015).
Mehra, V. et al. AKT inhibition generates potent polyfunctional clinical grade AUTO1 CAR T-cells, enhancing function and survival. J. Immunother. Cancer 11 , e007002 (2023).
Nagashima, T. et al. Discovery of novel forkhead box O1 inhibitors for treating type 2 diabetes: improvement of fasting glycemia in diabetic db / db mice. Mol. Pharmacol. 78 , 961–970 (2010).
Staron, M. M. et al. The transcription factor FoxO1 sustains expression of the inhibitory receptor PD-1 and survival of antiviral CD8 + T cells during chronic infection. Immunity 41 , 802–814 (2014).
Delpoux, A. et al. Continuous activity of Foxo1 is required to prevent anergy and maintain the memory state of CD8 + T cells. J. Exp. Med. 215 , 575–594 (2018).
Delpoux, A., Lai, C.-Y., Hedrick, S. M. & Doedens, A. L. FOXO1 opposition of CD8 + T cell effector programming confers early memory properties and phenotypic diversity. Proc. Natl Acad. Sci. USA 114 , E8865–E8874 (2017).
Delpoux, A. et al. FOXO1 constrains activation and regulates senescence in CD8 T cells. Cell Rep. 34 , 108674 (2021).
Lynn, R. C. et al. c-Jun overexpression in CAR T cells induces exhaustion resistance. Nature 576 , 293–300 (2019).
Weber, E. W. et al. Pharmacologic control of CAR-T cell function using dasatinib. Blood Adv. 3 , 711–717 (2019).
Im, S. J. et al. Defining CD8 + T cells that provide the proliferative burst after PD-1 therapy. Nature 537 , 417–421 (2016).
Siddiqui, I. et al. Intratumoral Tcf1 + PD-1 + CD8 + T Cells with stem-like properties promote tumor control in response to vaccination and checkpoint blockade immunotherapy. Immunity 50 , 195–211 (2019).
Shan, Q. et al. Ectopic Tcf1 expression instills a stem-like program in exhausted CD8 + T cells to enhance viral and tumor immunity. Cell. Mol. Immunol. 18 , 1262–1277 (2021).
Wu, T. et al. The TCF1–Bcl6 axis counteracts type I interferon to repress exhaustion and maintain T cell stemness. Sci. Immunol. 1 , eaai8593 (2016).
Tsui, C. et al. MYB orchestrates T cell exhaustion and response to checkpoint inhibition. Nature 609 , 354–360 (2022).
Wang, Y. et al. The transcription factor TCF1 preserves the effector function of exhausted CD8 T cells during chronic viral infection. Front. Immunol. 10 , 169 (2019).
Giuffrida, L. et al. IL-15 preconditioning augments CAR T cell responses to checkpoint blockade for improved treatment of solid tumors. Mol. Ther. 28 , 2379–2393 (2020).
Hudson, W. H. et al. Proliferating transitory T Cells with an effector-like transcriptional signature emerge from PD-1 + stem-like CD8 + T cells during chronic infection. Immunity 51 , 1043–1058 (2019).
Gattinoni, L. et al. Wnt signaling arrests effector T cell differentiation and generates CD8 + memory stem cells. Nat. Med. 15 , 808–813 (2009).
Kerdiles, Y. M. et al. Foxo transcription factors control regulatory T cell development and function. Immunity 33 , 890–904 (2010).
Ouyang, W. et al. Novel Foxo1-dependent transcriptional programs control T reg cell function. Nature 491 , 554–559 (2012).
Shan, Q. et al. Tcf1 and Lef1 provide constant supervision to mature CD8 + T cell identity and function by organizing genomic architecture. Nat. Commun. 12 , 5863 (2021).
Hatta, M. & Cirillo, L. A. Chromatin opening and stable perturbation of core histone:DNA contacts by FoxO1. J. Biol. Chem. 282 , 35583–35593 (2007).
Miller, B. C. et al. Subsets of exhausted CD8 + T cells differentially mediate tumor control and respond to checkpoint blockade. Nat. Immunol. 20 , 326–336 (2019).
Chen, Z. et al. TCF-1-centered transcriptional network drives an effector versus exhausted CD8 T cell-fate decision. Immunity 51 , 840–855 (2019).
Farber, D. L., Yudanin, N. A. & Restifo, N. P. Human memory T cells: generation, compartmentalization and homeostasis. Nat. Rev. Immunol. 14 , 24–35 (2014).
Matsuzaki, H. et al. Acetylation of Foxo1 alters its DNA-binding ability and sensitivity to phosphorylation. Proc. Natl Acad. Sci. USA 102 , 11278–11283 (2005).
Miyara, M. et al. Functional delineation and differentiation dynamics of human CD4 + T cells expressing the FoxP3 transcription factor. Immunity 30 , 899–911 (2009).
Bonacci, B. et al. Requirements for growth and IL-10 expression of highly purified human T regulatory cells. J. Clin. Immunol. 32 , 1118–1128 (2012).
Johnson, J. L. et al. Lineage-determining transcription factor TCF-1 initiates the epigenetic identity of T cells. Immunity 48 , 243–257 (2018).
Wang, W. et al. TCF-1 promotes chromatin interactions across topologically associating domains in T cell progenitors. Nat. Immunol. 23 , 1052–1062 (2022).
Gray, S. M., Amezquita, R. A., Guan, T., Kleinstein, S. H. & Kaech, S. M. Polycomb repressive complex 2-mediated chromatin repression guides effector CD8 + T cell terminal differentiation and loss of multipotency. Immunity 46 , 596–608 (2017).
Oh, H.-M. et al. STAT3 protein promotes T-cell survival and inhibits interleukin-2 production through up-regulation of Class O Forkhead transcription factors. J. Biol. Chem. 286 , 30888–30897 (2011).
Rao, R. R., Li, Q., Gubbels Bupp, M. R. & Shrikant, P. A. Transcription factor Foxo1 represses T-bet-mediated effector functions and promotes memory CD8 + T cell differentiation. Immunity 36 , 374–387 (2012).
Tejera, M. M., Kim, E. H., Sullivan, J. A., Plisch, E. H. & Suresh, M. FoxO1 controls effector-to-memory transition and maintenance of functional CD8 T cell memory. J. Immunol. 191 , 187–199 (2013).
Ouyang, W., Beckett, O., Flavell, R. A. & Li, M. O. An essential role of the Forkhead-box transcription factor Foxo1 in control of T cell homeostasis and tolerance. Immunity 30 , 358–371 (2009).
Zhang, L. et al. Mammalian target of rapamycin complex 2 controls CD8 T cell memory differentiation in a Foxo1-dependent manner. Cell Rep. 14 , 1206–1217 (2016).
Smole, A. et al. Expression of inducible factors reprograms CAR-T cells for enhanced function and safety. Cancer Cell 40 , 1470–1487 (2022).
Moffett, H. F. et al. Hit-and-run programming of therapeutic cytoreagents using mRNA nanocarriers. Nat. Commun. 8 , 389 (2017).
Roth, T. L. et al. Pooled knockin targeting for genome engineering of cellular immunotherapies. Cell 181 , 728–744 (2020).
Andreatta, M. et al. Interpretation of T cell states from single-cell transcriptomics data using reference atlases. Nat. Commun. 12 , 2965 (2021).
Jung, I.-Y. et al. BLIMP1 and NR4A3 transcription factors reciprocally regulate antitumor CAR T cell stemness and exhaustion. Sci. Transl. Med. 14 , eabn7336 (2022).
Hao, Y. et al. Integrated analysis of multimodal single-cell data. Cell 184 , 3573–3587 (2021).
Bukhari, S. et al. Single-cell RNA sequencing reveals distinct T cell populations in immune-related adverse events of checkpoint inhibitors. Cell Rep. Med. 4 , 100868 (2023).
Weber, E .W. Weber Lab. GitHub https://github.com/Weber-Lab-CHOP/FOXO1_2024 (2024).
Jung, I.-Y. et al. Type I interferon signaling via the EGR2 transcriptional regulator potentiates CAR T cell-intrinsic dysfunction. Cancer Discov. 13 , 1636–1655 (2023).
Download references
Acknowledgements
We thank W. Yu for guidance with single-cell ATAC-seq data analysis; R. Majzner and F. Staback Rodriguez for thoughtful discussions; the National Cancer Institute at Frederick for providing the 1A7 anti-14g2a idiotype antibody; B. Jena and L. J. N. Cooper for providing the monoclonal anti-FMC63 idiotype antibody; and the High Throughput Sequencing Core at CHOP for help with sequencing. We also acknowledge the Flow Cytometry Core at CHOP and the Stanford Institute for Stem Cell Biology and Regenerative Medicine FACS core for equipment and technical support. Schematics were created with BioRender.com. This work was supported by the National Cancer Institute Immunotherapy Discover and Development (1U01CA232361-A1 to S.A.G. and E.W.W.; K08CA23188-01 and U01CA260852 to A.T.S.; and U54CA232568-01 to C.L.M.); the National Human Genome Research Institute (K99 HGHG012579 to C.A.L.); the Parker Institute for Cancer Immunotherapy (C.A.L., A.T.S., C.L.M. and E.W.W); the V Foundation for Cancer Research (E.W.W.); a Society for Immunotherapy of Cancer Rosenberg Scholar Award (E.W.W.); Stand Up 2 Cancer (St. Baldrick’s) (NCI SU2CAACR-DT1113 to C.L.M.); the Virginia and D.K. Ludwig Fund for Cancer Research (C.L.M.); and NCI U2C CA233285 (K.T.). C.L.M., A.T.S., C.A.L. and E.W.W. are members of the Parker Institute for Cancer Immunotherapy, which supports cancer immunotherapy research at Stanford University and the University of Pennsylvania. Stand Up 2 Cancer is a program of the Entertainment Industry Foundation administered by the American Association for Cancer Research.
Author information
Bence Daniel
Present address: Genentech, South San Francisco, CA, USA
These authors contributed equally: Alexander E. Doan, Katherine P. Mueller, Andy Y. Chen
These authors jointly supervised this work: Crystal L. Mackall, Evan W. Weber
Authors and Affiliations
Center for Cancer Cell Therapy, Stanford Cancer Institute, Stanford University School of Medicine, Stanford, CA, USA
Alexander E. Doan, John Lattin, Peng Xu, Dorota Klysz, Malek Bashti, Patrick J. Quinn, Elena Sotillo & Crystal L. Mackall
Department of Pediatrics, Perelman School of Medicine, University of Pennsylvania, Philadelphia, PA, USA
Katherine P. Mueller, Geoffrey T. Rouin, Yingshi Chen, Martina Markovska, Brett Mozarsky, Jose Arias-Umana, Robert Hapke, Alice Wang, Gabrielle Zuern, Faith Ryu, Junior Hall, Kai Tan, Stephan A. Grupp, Susan E. McClory & Evan W. Weber
Center for Cellular and Molecular Therapeutics, Children’s Hospital of Philadelphia, Philadelphia, PA, USA
Katherine P. Mueller, Geoffrey T. Rouin, Yingshi Chen, Martina Markovska, Brett Mozarsky, Jose Arias-Umana, Robert Hapke, Gabrielle Zuern, Faith Ryu & Evan W. Weber
Center for Cellular Immunotherapies, Perelman School of Medicine, University of Pennsylvania, Philadelphia, PA, USA
Katherine P. Mueller, Geoffrey T. Rouin, Yingshi Chen, Martina Markovska, Brett Mozarsky, Jose Arias-Umana, Robert Hapke, Gabrielle Zuern, Gregory M. Chen, Faith Ryu, Meghan Logun, Joseph A. Fraietta & Evan W. Weber
Department of Pathology, Stanford University, Stanford, CA, USA
Andy Y. Chen, Bence Daniel, Katalin Sandor, Wenxi Zhang, Caleb A. Lareau & Ansuman T. Satpathy
Department of Bioengineering, Stanford University, Stanford, CA, USA
Andy Y. Chen
Gladstone–UCSF Institute of Genomic Immunology, San Francisco, CA, USA
Center for Personal Dynamic Regulomes, Stanford University, Stanford, CA, USA
Department of Genetics, Stanford University, Stanford, CA, USA
Bence Daniel & Zhuang Miao
Department of Microbiology, Perelman School of Medicine, University of Pennsylvania, Philadelphia, PA, USA
In-Young Jung & Joseph A. Fraietta
Department of Pathology and Laboratory Medicine, Perelman School of Medicine, University of Pennsylvania, Philadelphia, PA, USA
Gregory M. Chen & Joseph A. Fraietta
Department of Neurosurgery, Perelman School of Medicine, University of Pennsylvania, Philadelphia, PA, USA
Meghan Logun
Center for Childhood Cancer Research, Children’s Hospital of Philadelphia, Philadelphia, PA, USA
Junior Hall, Kai Tan, Stephan A. Grupp, Susan E. McClory & Evan W. Weber
Abramson Cancer Center, Perelman School of Medicine, University of Pennsylvania, Philadelphia, PA, USA
Junior Hall, Kai Tan, Stephan A. Grupp, Joseph A. Fraietta & Evan W. Weber
Parker Institute for Cancer Immunotherapy, San Francisco, CA, USA
Caleb A. Lareau, Ansuman T. Satpathy, Crystal L. Mackall & Evan W. Weber
Department of Pediatrics, Stanford University, Stanford, CA, USA
Crystal L. Mackall
Department of Medicine, Stanford University, Stanford, CA, USA
You can also search for this author in PubMed Google Scholar
Contributions
E.W.W. and C.L.M. conceived the study, secured funding and supervised the project. A.E.D., K.P.M., G.T.R., B.D., J.L., Y.C., B.M., M.M., J.A.-U., R.H., A.W., P.X., D.K., G.Z., M.B., P.J.Q., Z.M., K.S., W.Z., F.R., M.L., J.H., S.E.M. and E.W.W. designed and performed wet-lab experiments. K.P.M., A.Y.C., B.D., A.W., G.M.C., and C.A.L. performed RNA-seq and ATAC-seq computational analyses. I.-Y.J. and J.A.F. performed analyses on and interpreted clinical data. K.T., S.A.G., J.A.F., E.S. and A.T.S. supervised experiments and analyses. A.E.D., K.P.M., E.S., C.L.M. and E.W.W. wrote the manuscript. All authors discussed the results and edited the manuscript.
Corresponding authors
Correspondence to Crystal L. Mackall or Evan W. Weber .
Ethics declarations
Competing interests.
C.A.L. is a consultant to Cartography Biosciences. S.A.G. receives research funding from Novartis, Kite, Vertex and Servier; consults for Novartis, Roche, GSK, Humanigen, CBMG, Eureka, Janssen/JNJ and Jazz Pharmaceuticals; and has advised for Novartis, Adaptimmune, TCR2, Cellctis, Juno, Vertex, Allogene, Jazz Pharmaceuticals and Cabaletta. J.A.F. receives research funding from Tceleron (formerly Tmunity Therapeutics) and Danaher Corporation; consults for Retro Biosciences; and is a member of the scientific advisory boards of Cartography Biosciences and Shennon Biotechnologies. A.T.S. is a founder of Immunai and Cartography Biosciences and receives research funding from Allogene Therapeutics and Merck Research Laboratories. C.L.M. is a co-founder of and holds equity in Link Cell Therapies, Cargo Therapeutics (formerly Syncopation Life Sciences) and Lyell Immunopharma; holds equity and consults for Mammoth and Ensoma; consults for Immatics and Nektar; and receives research funding from Tune Therapeutics. E.W.W. holds equity in Lyell Immunopharma and consults for Umoja Immunopharma. The remaining authors declare no competing interests.
Peer review
Peer review information.
Nature thanks the anonymous reviewer(s) for their contribution to the peer review of this work. Peer reviewer reports are available.
Additional information
Publisher’s note Springer Nature remains neutral with regard to jurisdictional claims in published maps and institutional affiliations.
Extended data figures and tables
Extended data fig. 1 pharmacological inhibition of foxo1 impairs expansion, formation of a memory phenotype and antitumour function in cd19.28ζ and cd19.bbζ car t cells..
CAR T cells were treated with DMSO or 10 nM or 100 nM of the small molecule AS1842856 (FOXO1 i ) starting on day 4 post-activation and treated every 2–3 days thereafter. a , Schematic of FOXO1 i experimental model. b , CD19.28ζ (left) or CD19.BBζ (right) CAR T cell expansion ( n = 2 donors). c , Percent CD8 + in CD19.28ζ (circles) and CD19.BBζ (squares) cells ( n = 2 donors for each CAR). d , Apoptosis in CD19.BBζ CAR T cells at day 15 post-activation. Contour plots show 1 representative donor and bar graphs show mean±s.e.m. of n = 3 donors. e , Expression of memory- and exhaustion-associated markers on CD19.28ζ and CD19.BBζ cells. Histograms show 1 representative donor ( n = 2 donors). f , Cytokine secretion from CD19.28ζ and CD19.BBζ cells in response to Nalm6 cells. Graphs show mean±s.d. of triplicate wells from 1 representative donor ( n = 2 donors). g , Cytotoxicity of CD19.BBζ cells against Nalm6 cells at a 1:1 E:T ratio. Data is normalized to t = 0 and show mean±s.d. of triplicate wells from 1 representative donor ( n = 2 donors). Statistics are shown for t = 60 h. Statistical comparisons were performed using paired two-tailed Student’s t -test ( c ), two-way ANOVA with Šídák’s test ( d ) and one-way ( f ) or two-way ( g ) ANOVA with Dunnett’s test. E:T ratio, effector:target cell ratio. NS, not significant.
Extended Data Fig. 2 CRISPR knockout of FOXO1 attenuates memory formation and promotes exhaustion in CD19.BBζ and HA.28ζ CAR T cells.
a – i , CRISPR–Cas9 gene editing of AAVS1 (AAVS1) or FOXO1 (FOXO1 KO ) in CD19.BBζ CAR T cells. a , Schematic depicting generation of FOXO1 KO CAR T cells and downstream assays. b , Day 14 FOXO1 KO expansion normalized to AAVS1. Data show mean±s.e.m. of n = 3 donors. c – f , Flow cytometric analysis of memory- and exhaustion-associated markers on CD8 + ( c , e ) and CD4 + ( d , f ) CD19.BBζ cells. Histograms and contour plots show a representative donor and bar graphs show mean±s.e.m. of n = 3-6 donors. CD62L, IL-7Rα, TCF1, and CD39 histograms in c also appear in Fig. 1c . g , MFI of CD62L in FOXO1 + and FOXO1 − gated subpopulations of CD19.BBζ cells. h , Schematic showing CD62L lo / FOXO1 KO cell negative selection strategy for RNA-seq experiments. i , GO term analyses showing curated lists of up- and downregulated processes in FOXO1 KO compared to AAVS1. Data show Benjamini–Hochberg-adjusted P value ( n = 3 donors). j , Flow cytometric analysis of memory- and exhaustion-associated markers in day 15 HA.28ζ CAR T cells. Background-subtracted MFI is displayed. k , Cytokine secretion from day 15 HA.28ζ cells in response to Nalm6. Graphs show mean±s.d. of 3 technical replicates from one representative donor ( n = 2 donors). Statistical comparisons were performed using paired two-tailed Student’s t -test ( b , c , d , g ), two-way ANOVA with Bonferroni’s test ( e , f ), two-tailed Student’s t -test ( k ) and one-sided hypergeometric test ( i ).
Extended Data Fig. 3 FOXO1 overexpression promotes a memory phenotype and mitigates exhaustion in CAR T cells.
a , Schematic depicting engineering of truncated NGFR-only (tNGFR), TCF1/tNGFR- (TCF1 OE ), and FOXO1/tNGFR- (FOXO1 OE ) CAR T cells and magnetic isolation of tNGFR + cells for downstream analyses. b – e , Phenotypic and functional analyses of CD19.BBζ CAR T cells at baseline and during repeat stimulation with Nalm6 cells. b , c , Flow cytometric analysis of CD62L and IL-7Rα ( b ) and TCF1 and LEF1 ( c ) from 1 representative donor ( n = 4 donors). d , Cytokine secretion from CD19.BBζ cells after 1 or 4 stimulations with Nalm6 cells. Data show mean ± s.d. of 2–3 triplicate wells from 1 representative donor ( n = 2 donors). e , Flow cytometric analysis of CD62L, IL-7Rα, and CD39 on tNGFR + CD8 + CAR T cells prior to the first stimulation (Stim 0) and 7 days after the third stimulation (Stim 3). Data show mean ± s.e.m. of mean fluorescence intensity normalized to tNGFR levels from n = 2–3 donors. f – i , CAR T cell exhaustion model 7 , 23 whereby T cells express a high-affinity GD2-targeting CAR (HA.28ζ) that promotes antigen-independent tonic CAR signalling. f , Model schematic. g , Flow cytometric analysis of day 15 CD62L and IL-7Rα. Data show 1 representative donor ( n = 5 donors). h , Cytotoxicity of day 15 HA.28ζ cells against 143B cells at a 1:8 E:T ratio. Data is normalized to t = 0 and show mean±s.d. of 3 triplicate wells from 1 representative donor ( n = 3 donors). Statistics were performed at t = 96 h. i , j , Seahorse metabolic analyses on day 13 of culture ( n = 2 donors). i , Ratio of OCR to ECAR of HA.28ζ cells. j , CD19.28ζ cell OCR (left), OCR to ECAR ratio (centre), and spare respiratory capacity (right). OCR line graph shows 1 representative donor. Bar graphs show mean ± s.d. of three representative time points within each donor. Statistical comparisons were performed using one-way ANOVA with Tukey’s test ( h ) or Dunnett’s test ( i , j ). E:T ratio, effector:target cell ratio. OCR, Oxygen consumption rate. ECAR, extracellular acidification rate.
Extended Data Fig. 4 Overexpression of FOXO1 induces a memory-like transcriptional program in CAR T cells.
a – g , Bulk RNA-seq analyses of day 15 tNGFR + CD4 + HA.28ζ CAR T cells overexpressing tNGFR, TCF1 OE , or FOXO1 OE ( n = 3 donors). a , PCA of CD4 + cells. b , PCA that includes CD4 + samples plotted in a and CD8 + samples plotted in Fig. 2a . c , Venn diagram showing the number of unique and shared DEGs in CD4 + TCF1 OE and FOXO1 OE cells compared to tNGFR cells (Bonferroni-adjusted P < 0.05 with abs(log 2 FC)>0.5). d , Expression of memory- and exhaustion-associated genes. Centre line represents the mean counts per million. e , Heat map and hierarchical clustering of DEGs. Genes of interest are shown. Scale shows normalized z-scores for each DEG. f , GSVA using published human CD4 + regulatory T cell (T reg ) signatures 42 , 43 . Centre line represents mean score. g , GO term analyses showing curated lists of top up- and downregulated processes in CD4 + FOXO1 OE and TCF1 OE cells versus tNGFR cells. Data show Benjamini–Hochberg-adjusted P . h , QIAGEN IPA of upregulated and downregulated TF pathways in FOXO1 OE cells versus tNGFR cells. Data show adjusted P . i – k , Bulk RNA-seq analyses of day 15 tNGFR + CD8 + CD19.28ζ cells overexpressing tNGFR, TCF1 OE , or FOXO1 OE ( n = 3 donors). i , PCA analysis. j , Venn diagram showing the number of unique and shared DEGs in TCF1 OE and FOXO1 OE cells compared to tNGFR cells (Bonferroni-adjusted P < 0.05 with log 2 (fold change) < 0.5). k , Heat map and hierarchical clustering of DEGs. Genes of interest are shown. Scale shows normalized z-scores for each DEG. Statistical comparisons were performed using DESeq2 ( c , d , e , j , k ), repeated-measures one-way ANOVA with Tukey’s test ( f ) and one-sided hypergeometric test ( g ). NS, not significant.
Extended Data Fig. 5 FOXO1 or TCF1 overexpression induces chromatin remodelling in CD19.28ζ and HA.28ζ CAR T cells.
a – e , Bulk ATAC-seq analyses of day 15 tNGFR + CD8 + CAR T cells expressing either CD19.28ζ ( a – d ) or HA.28ζ ( e ) ( n = 3 donors). a , PCA of CD19.28ζ cells. b , c , Rank-ordered plot of differentially accessible TF-binding motifs ( P < 0.05 with abs(log 2 FC)>0.5) in FOXO1 OE cells ( b ) and TCF1 OE cells ( c ) versus tNGFR cells. d , e , Heat maps and hierarchical clustering of mean differential motif accessibility of CD19.28ζ ( d ) or HA.28ζ ( e ) cells. Scales show normalized z-scores for each motif. Statistical comparisons were performed using DESeq2 ( b – e ).
Extended Data Fig. 6 Nuclear-restricted FOXO1 promotes a memory-like phenotype but impairs effector function.
a , Schematic showing a mutated variant of FOXO1 that contains three amino acid substitutions (T24A, S256A, and S319A) which restrict nuclear export (FOXO1 3A ). b , Analysis of soluble and chromatin-bound FOXO1 fractions isolated from tNGFR + non-CAR T cells that were activated with Dynabeads for 24 h prior to cell collection. Western blots (left) and bar graph (right) representing the ratio of chromatin-bound to soluble FOXO1 normalized to mock T cells are shown for 1 representative donor ( n = 2 donors). c , FOXO1 expression in CD19.28ζ and HA.28ζ CAR T cells from 1 representative donor ( n = 5 donors). d , CD62L and IL-7Rα expression in CD19.28ζ and HA.28ζ CAR T cells from 1 representative donor ( n = 3 donors). e , TCF1 and LEF1 expression in CD19.28ζ CAR T cells from 1 representative donor ( n = 3 donors). f , g , RNA-seq on HA.28ζ CAR T cells. tNGFR and FOXO1 OE samples are also represented in Fig. 2 and Extended Data Fig. 4 . f , PCA. g , GO term analyses showing curated lists of top up- and downregulated processes in FOXO1 3A vs FOXO1 OE . Data show Benjamini–Hochberg-adjusted P value. h , Cytotoxicity of HA.28ζ cells against Nalm6 at a 1:1 E:T ratio. Data are normalized to t = 0 and show mean±s.d. from 1 representative donor ( n = 3 donors). Statistics were performed at t = 96 h. i , Cytokine secretion from day 15 HA.28ζ CAR T cells in response to 143B cells. Plots show mean±s.d. of 3 wells from 1 representative donor ( n = 3 donors). Statistical comparisons were performed using one-sided hypergeometric test ( g ), one-way ANOVA with Tukey’s test ( h ) or Dunnett’s test ( i ). E:T ratio, effector:target cell ratio.
Extended Data Fig. 7 FOXO1 OE CAR T cells show enhanced antitumour activity in leukaemia xenograft models .
a , b , A curative dose of 2×10 6 tNGFR + CD19.BBζ CAR T cells overexpressing tNGFR, TCF1 OE , FOXO1 OE , or FOXO1 3A were infused into Nalm6 leukaemia-bearing mice 7 days post-engraftment ( n = 2 donors tested in 2 independent experiments) a , Experimental schematic (left) and tumour bioluminescence of multiple time points (right) from 1 representative donor ( n = 3-5 mice per group). b , Tumour bioluminescence from day 42-45. Data show mean±s.e.m. from 2 donors tested in 2 independent experiments ( n = 3-10 mice per group; n = 1 donor for FOXO1 3A ). c – g , A curative dose of 1×10 6 tNGFR + CD19.28ζ cells were infused into Nalm6-bearing mice 7 days post-engraftment. Mice were rechallenged with 10×10 6 CD19 + or CD19 − Nalm6 on day 21 post-CAR T cell infusion ( n = 2 donors tested in 2 independent experiments). c , Tumour bioluminescence over time. Data show mean±s.e.m. of n = 3–7 mice per group from 1 representative donor. d , CD19.28ζ and tNGFR expression on circulating CD45 + CAR T cells on day 21. Contour plots show 1 representative mouse from each condition from 1 representative donor. e , Quantification of circulating CD45 + CAR T cells on days 7, 21, and 28. f , CD4 + and CD8 + CAR T cells on day 7 (data derived from e ). g , Percent CD8 + CAR T cells. Graphs in e – g show mean±s.e.m. of n = 3–7 mice per group from 1 representative donor. Statistical comparisons were performed using nonparametric two-tailed Mann–Whitney test ( b ) and two-way ( c ) and one-way ANOVA with Dunnett’s test ( e , f ) and mixed-effects model with Dunnett’s test ( g ). NS, not significant.
Extended Data Fig. 8 FOXO1 OE reprogramming and enhanced antitumour activity are dependent on DNA binding.
a , Schematic depicting construct design and amino acid substitutions (K245A and K248A) to generate human FOXO1 DBD (top) and western blots of indicated proteins in soluble and chromatin-bound fractions isolated from day 8 tNGFR + CD19.28ζ CAR T cells (bottom). Densitometry analyses are displayed below the blots. 1 representative donor from n = 2 donors. b , FOXO1 expression in CD19.28ζ CAR T cells from one representative donor ( n = 5 donors). c , Bulk RNA-seq analyses of day 15 tNGFR + CD8 + HA.28ζ CAR T cells show unique and shared DEGs in FOXO1 DBD and FOXO1 OE compared with tNGFR (Bonferroni-adjusted P < 0.05 with abs(log 2 FC)>0.5). FOXO1 OE samples are also represented in Fig. 2 and Extended Data Figs. 4 and 6 . d , Bulk ATAC-seq of day 15 tNGFR + CD8 + HA.28ζ CAR T cells. Rank-ordered plot of differentially accessible TF-binding motifs in FOXO1 OE cells versus FOXO1 DBD cells ( P < 0.05 with abs(log 2 FC)>0.5). FOXO1 OE samples are also represented in Fig. 2 and Extended Data Fig. 5 . e , Schematic of stress test model (left) whereby Nalm6-engrafted mice were treated with mock T cells or FOXO1 OE or FOXO1 DBD CD19.28ζ CAR T cells. Survival curve shows pooled data from 2 donors tested in 2 independent experiments ( n = 10 mice per group, FOXO1 OE data from 1 donor are also represented in Fig. 3a ). f , TCF7 knockout efficiency for bulk RNA-seq data corresponding to Fig. 3f,g . Data show the mean of n = 3 donors with 2 technical replicates per donor. Statistical comparisons were performed using DESeq2 ( c , d ) and log-rank Mantel–Cox test ( e ).
Extended Data Fig. 9 FOXO1 OE CAR T cells exhibit improved persistence and effector- and tissue-residence-associated transcriptomic signatures in a solid-tumour xenograft model.
5×10 6 Her2.BBζ CAR T cells were infused into 143B-bearing mice 3 days post-engraftment. Tumours and spleens were collected on day 29 post-engraftment for phenotypic, functional, and sequencing-based assays. a , Total splenic CAR T cells. b , Total CD4 + (left) and CD8 + (right) splenic CAR T cells. c , Ratio of CD8 + to CD4 + tumour-infiltrating CAR T cells from donor 1 ( n = 3 mice per group). Donor 2 is shown in Fig. 4d . d , Ratio of CD8 + to CD4 + CAR T cells from spleens. Data in a – d show mean±s.d. of n = 13 mice per group from 2 donors tested in 2 independent experiments unless otherwise stated. e – i , Single-cell RNA-seq on day 29 tumour-infiltrating FOXO1 OE or tNGFR cells. Cells were sorted and pooled from n = 5 mice per group from 1 donor. e , Top enriched GO terms in Cluster 1, which was biased towards FOXO1 OE cells. Gene ratio and Benjamini–Hochberg-adjusted P value are shown. f , T reg transcriptional signature 58 score. g , T eff signature genes corresponding to T eff scores in Fig. 4i . h , T RM signature genes corresponding to T RM scores in Fig. 4i . i , T reg signature genes corresponding to scores in f . Statistical comparisons were performed using two-tailed Student’s t -test ( a-d ), one-sided hypergeometric test ( e ) and two-sided Wilcoxon rank-sum test ( f ).
Extended Data Fig. 10 Endogenous TCF7 transcript and FOXO1 regulon, but not TCF OE transcriptional or epigenetic signatures, predict CAR T cell and TIL responses in patients.
a , ssGSEA on RNA-seq from CAR-stimulated CTL019 cells 2 (complete responder, CR, partial responder with transformed disease, PR TD , n = 3; partial responder, PR, n = 5; non-responder, NR, n = 21). Enrichment score stratification points for patient survival analyses were determined using previously published methods 60 . a , TCF7 expression is shown for patient outcomes (left) and overall survival (right). b – d , P values (top) and hazard ratios (bottom) of different stratification points in relation to overall survival (OS) of TCF7 expression ( b ), FOXO1 expression ( c ) and FOXO1 regulon ( d ). Dotted lines are drawn at P < 0.05 and black arrows indicate the stratification points used. e , An epigenetic signatured derived from CD8 + CD19.28ζ FOXO1 OE bulk ATAC-seq data was applied to pre-manufactured paediatric CAR T cell single-cell ATAC-seq data 5 . Violin plots show TCF1 OE epigenetic signature scores for patients with durable (Patient 52, n = 616 cells; Patient 54, n = 2959 cells) and short (Patient 38, n = 2093 cells; Patient 66, n = 2355 cells) CAR T cell persistence. f , GSEA was performed with CD8 + HA.28ζ TCF1 OE DEGs and DEGs derived from CD39 − CD69 − patient TILs in adult melanoma 8 . Violin plots in a , e show minima and maxima; centre lines represent mean; dashed lines represent top and bottom quartiles. Statistical comparisons were performed using two-tailed Mann–Whitney test ( a , left), log-rank Mantel–Cox test ( a , right), two-sided Wald test ( e ), and two-sided Kolmogorov–Smirnov test ( f ).
Supplementary information
Supplementary figures.
This file includes Supplementary Fig. 1 - the representative flow cytometry gating strategy, Supplementary Fig. 2 - bulk ATAC-seq quality control metrics and Supplementary Fig. 3 - raw western blot data corresponding to Extended Data Figs 6b and 8a.
Reporting Summary
Supplementary table 1.
FOXO1 regulon genes.
Supplementary Table 2
Reagents used in this study.
Peer Review File
Source data, source data fig. 1, source data fig. 2, source data fig. 3, source data fig. 4, source data fig. 5, source data extended data fig. 1, source data extended data fig. 2, source data extended data fig. 3, source data extended data fig. 4, source data extended data fig. 6, source data extended data fig. 7, source data extended data fig. 8, source data extended data fig. 9, source data extended data fig. 10, rights and permissions.
Open Access This article is licensed under a Creative Commons Attribution 4.0 International License, which permits use, sharing, adaptation, distribution and reproduction in any medium or format, as long as you give appropriate credit to the original author(s) and the source, provide a link to the Creative Commons licence, and indicate if changes were made. The images or other third party material in this article are included in the article’s Creative Commons licence, unless indicated otherwise in a credit line to the material. If material is not included in the article’s Creative Commons licence and your intended use is not permitted by statutory regulation or exceeds the permitted use, you will need to obtain permission directly from the copyright holder. To view a copy of this licence, visit http://creativecommons.org/licenses/by/4.0/ .
Reprints and permissions
About this article
Cite this article.
Doan, A.E., Mueller, K.P., Chen, A.Y. et al. FOXO1 is a master regulator of memory programming in CAR T cells. Nature (2024). https://doi.org/10.1038/s41586-024-07300-8
Download citation
Received : 12 April 2023
Accepted : 12 March 2024
Published : 10 April 2024
DOI : https://doi.org/10.1038/s41586-024-07300-8
Share this article
Anyone you share the following link with will be able to read this content:
Sorry, a shareable link is not currently available for this article.
Provided by the Springer Nature SharedIt content-sharing initiative
This article is cited by
How to supercharge cancer-fighting cells: give them stem-cell skills.
- Sara Reardon
Nature (2024)
By submitting a comment you agree to abide by our Terms and Community Guidelines . If you find something abusive or that does not comply with our terms or guidelines please flag it as inappropriate.
Quick links
- Explore articles by subject
- Guide to authors
- Editorial policies
Sign up for the Nature Briefing newsletter — what matters in science, free to your inbox daily.


An official website of the United States government
The .gov means it’s official. Federal government websites often end in .gov or .mil. Before sharing sensitive information, make sure you’re on a federal government site.
The site is secure. The https:// ensures that you are connecting to the official website and that any information you provide is encrypted and transmitted securely.
- Publications
- Account settings
Preview improvements coming to the PMC website in October 2024. Learn More or Try it out now .
- Advanced Search
- Journal List
- HHS Author Manuscripts

The benefits and risks of stem cell technology
The potential impact of stem cell technology on medical and dental practice is vast. Stem cell research will not only provide the foundation for future therapies, but also reveal unique insights into basic disease mechanisms. Therefore, an understanding of stem cell technology will be necessary for clinicians in the future. Herein, we give a basic overview of stem cell biology and therapeutics for the practicing clinician.
Introduction
Stem cell technology will transform medical practice. While stem cell research has already elucidated many basic disease mechanisms, the promise of stem cell–based therapies remains largely unrealized. In this review, we begin with an overview of different stem cell types. Next, we review the progress in using stem cells for regenerative therapy. Last, we discuss the risks associated with stem cell–based therapies.
Stem cell types
There are three major types of stem cells as follows: adult stem cells (also called tissue-specific stem cells), embryonic stem (ES) cells, and induced pluripotent stem (iPS) cells.
Adult stem cells
A majority of adult stem cells are lineage-restricted cells that often reside within ‘niches’ of their tissue of origin. Adult stem cells are characterized by their capacity for self-renewal and differentiation into tissue-specific cell types. Many adult tissues contain stem cells including skin, muscle, intestine, and bone marrow ( Gan et al , 1997 ; Artlett et al , 1998 ; Matsuoka et al , 2001 ; Coulombel, 2004 ; Humphries et al , 2011 ). However, it remains unclear whether all adult organs contain stem cells. Adult stem cells are quiescent but can be induced to replicate and differentiate after tissue injury to replace cells that have died. The process by which this occurs is poorly understood. Importantly, adult stem cells are exquisitely tissue-specific in that they can only differentiate into the mature cell type of the organ within which they reside ( Rinkevich et al , 2011 ).
Thus far, there are few accepted adult stem cell–based therapies. Hematopoietic stem cells (HSCs) can be used after myeloablation to repopulate the bone marrow in patients with hematologic disorders, potentially curing the underlying disorder ( Meletis and Terpos, 2009 ; Terwey et al , 2009 ; Casper et al , 2010 ; Hill and Copelan, 2010 ; Hoff and Bruch-Gerharz, 2010 ; de Witte et al , 2010 ). HSCs are found most abundantly in the bone marrow, but can also be harvested at birth from umbilical cord blood ( Broxmeyer et al , 1989 ). Similar to the HSCs harvested from bone marrow, cord blood stem cells are tissue-specific and can only be used to reconstitute the hematopoietic system ( Forraz et al , 2002 ; McGuckin et al , 2003 ; McGuckin and Forraz, 2008 ). In addition to HSCs, limbal stem cells have been used for corneal replacement ( Rama et al , 2010 ).
Mesenchymal stem cells (MSCs) are a subset of adult stem cells that may be particularly useful for stem cell–based therapies for three reasons. First, MSCs have been isolated from a variety of mesenchymal tissues, including bone marrow, muscle, circulating blood, blood vessels, and fat, thus making them abundant and readily available ( Deans and Moseley, 2000 ; Zhang et al , 2009 ; Lue et al , 2010 ; Portmann-Lanz et al , 2010 ). Second, MSCs can differentiate into a wide array of cell types, including osteoblasts, chondrocytes, and adipocytes ( Pittenger et al , 1999 ). This suggests that MSCs may have broader therapeutic applications compared to other adult stem cells. Third, MSCs exert potent paracrine effects enhancing the ability of injured tissue to repair itself. In fact, animal studies suggest that this may be the predominant mechanism by which MSCs promote tissue repair. The paracrine effects of MSC-based therapy have been shown to aid in angiogenic, antiapoptotic, and immunomodulatory processes. For instance, MSCs in culture secrete hepatocyte growth factor (HGF), insulin-like growth factor-1 (IGF-1), and vascular endothelial growth factor (VEGF) ( Nagaya et al , 2005 ). In a rat model of myocardial ischemia, injection of human bone marrow-derived stem cells upregulated cardiac expression of VEGF, HGF, bFGF, angiopoietin-1 and angiopoietin-2, and PDGF ( Yoon et al , 2005 ). In swine, injection of bone marrow-derived mononuclear cells into ischemic myocardium was shown to increase the expression of VEGF, enhance angiogenesis, and improve cardiac performance ( Tse et al , 2007 ). Bone marrow-derived stem cells have also been used in a number of small clinical trials with conflicting results. In the largest of these trials (REPAIR-AMI), 204 patients with acute myocardial infarction were randomized to receive bone marrow-derived progenitor cells vs placebo 3–7 days after reperfusion. After 4 months, the patients that were infused with stem cells showed improvement in left ventricular function compared to control patients. At 1 year, the combined endpoint of recurrent ischemia, revascularization, or death was decreased in the group treated with stem cells ( Schachinger et al , 2006 ).
Embryonic stem cells are derived from the inner cell mass of the developing embryo during the blastocyst stage ( Thomson et al , 1998 ). In contrast to adult stem cells, ES cells are pluripotent and can theoretically give rise to any cell type if exposed to the proper stimuli. Thus, ES cells possess a greater therapeutic potential than adult stem cells. However, four major obstacles exist to implementing ES cells therapeutically. First, directing ES cells to differentiate into a particular cell type has proven to be challenging. Second, ES cells can potentially transform into cancerous tissue. Third, after transplantation, immunological mismatch can occur resulting in host rejection. Fourth, harvesting cells from a potentially viable embryo raises ethical concerns. At the time of this publication, there are only two ongoing clinical trials utilizing human ES-derived cells. One trial is a safety study for the use of human ES-derived oligodendrocyte precursors in patients with paraplegia (Genron based in Menlo Park, California). The other is using human ES-derived retinal pigmented epithelial cells to treat blindness resulting from macular degeneration (Advanced Cell Technology, Santa Monica, CA, USA).
In stem cell research, the most exciting recent advancement has been the development of iPS cell technology. In 2006, the laboratory of Shinya Yamanaka at the Gladstone Institute was the first to reprogram adult mouse fibroblasts into an embryonic-like cell, or iPS cell, by overexpression of four transcription factors, Oct3/4, Sox2, c-Myc, and Klf4 under ES cell culture conditions ( Takahashi and Yamanaka, 2006 ). Yamakana's pioneering work in cellular reprogramming using adult mouse cells set the foundation for the successful creation of iPS cells from adult human cells by both his team ( Takahashi et al , 2007 ) and a group led by James Thomson at the University of Wisconsin ( Yu et al , 2007 ). These initial proof of concept studies were expanded upon by leading scientists such as George Daley, who created the first library of disease-specific iPS cell lines ( Park et al , 2008 ). These seminal discoveries in the cellular reprogramming of adult cells invigorated the stem cell field and created a niche for a new avenue of stem cell research based on iPS cells and their derivatives. Since the first publication on cellular reprogramming in 2006, there has been an exponential growth in the number of publications on iPS cells.
Similar to ES cells, iPS cells are pluripotent and, thus, have tremendous therapeutic potential. As of yet, there are no clinical trials using iPS cells. However, iPS cells are already powerful tools for modeling disease processes. Prior to iPS cell technology, in vitro cell culture disease models were limited to those cell types that could be harvested from the patient without harm – usually dermal fibroblasts from skin biopsies. However, mature dermal fibroblasts alone cannot recapitulate complicated disease processes involving multiple cell types. Using iPS technology, dermal fibroblasts can be de-differentiated into iPS cells. Subsequently, the iPS cells can be directed to differentiate into the cell type most beneficial for modeling a particular disease process. Advances in the production of iPS cells have found that the earliest pluripotent stage of the derivation process can be eliminated under certain circumstances. For instance, dermal fibroblasts have been directly differentiated into dopaminergic neurons by viral co-transduction of forebrain transcriptional regulators (Brn2, Myt1l, Zic1, Olig2, and Ascl1) in the presence of media containing neuronal survival factors [brain-derived neurotrophic factor, neurotrophin-3 (NT3), and glial-conditioned media] ( Qiang et al , 2011 ). Additionally, dermal fibroblasts have been directly differentiated into cardiomyocyte-like cells using the transcription factors Gata4, Mef2c, and Tb×5 ( Ieda et al , 2010 ). Regardless of the derivation process, once the cell type of interest is generated, the phenotype central to the disease process can be readily studied. In addition, compounds can be screened for therapeutic benefit and environmental toxins can be screened as potential contributors to the disease. Thus far, iPS cells have generated valuable in vitro models for many neurodegenerative (including Parkinson's disease, Huntington's disease, and amyotrophic lateral sclerosis), hematologic (including Fanconi's anemia and dyskeratosis congenital), and cardiac disorders (most notably the long QT syndrome) ( Park et al , 2008 ). iPS cells from patients with the long QT syndrome are particularly interesting as they may provide an excellent platform for rapidly screening drugs for a common, lethal side effect ( Zwi et al , 2009 ; Malan et al , 2011 ; Tiscornia et al , 2011 ). The development of patient-specific iPS cells for in vitro disease modeling will determine the potential for these cells to differentiate into desired cell lineages, serve as models for investigating the mechanisms underlying disease pathophysiology, and serve as tools for future preclinical drug screening and toxicology studies.
Regenerative therapy
Cardiovascular.
Despite substantial improvements in therapy, cardiovascular disease remains the leading cause of death in the industrialized world. Therefore, there is a particular interest in cardiovascular regenerative therapies. The potential of diverse progenitor cells to repair damaged heart tissue includes replacement (tissue transplant), restoration (activation of resident cardiac progenitor cells, paracrine effects), and regeneration (stem cell engraftment forming new myocytes) ( Codina et al , 2010 ). It is unclear whether the heart contains resident stem cells. However, experiments show that bone marrow mononuclear cells (BMCs) can repair myocardial damage, reduce left ventricular remodeling, and improve heart function by myocardial regeneration ( Hakuno et al , 2002 ; Amado et al , 2005 ; Dai et al , 2005 ; Schneider et al , 2008 ). The regenerative capacity of human heart tissue was further supported by the detection of the renewal of human cardiomyocytes (1% annually at the age of 25) by analysis of carbon-14 integration into human cardiomyocyte DNA ( Bergmann et al , 2009 ). It is not clear whether cardiomyocyte renewal is derived from resident adult stem cells, cardiomyocyte duplication, or homing of non-myocardial progenitor cells. Bone marrow cells home to the injured myocardium as shown by Y chromosome-positive BMCs in female recipients ( Deb et al , 2003 ). On the basis of these promising results, clinical trials in patients with ischemic heart disease have been initiated primarily using bone marrow-derived cells. However, these small trials have shown controversial results. This is likely due to a lack of standardization for cell harvesting and delivery procedures. This highlights the need for a better understanding of the basic mechanisms underlying stem cell isolation and homing prior to clinical implementation.
Although stem cells have the capacity to differentiate into neurons, oligodendrocytes, and astrocytes, novel clinical stem cell–based therapies for central and peripheral nervous system diseases have yet to be realized. It is widely hoped that transplantation of stem cells will provide effective therapy for Parkinson's disease, Alzheimer's disease, Huntington's Disease, amyloid lateral sclerosis, spinal cord injury, and stroke. Several encouraging animal studies have shown that stem cells can rescue some degree of neurological function after injury ( Daniela et al , 2007 ; Hu et al , 2010 ; Shimada and Spees, 2011 ). Currently, a number of clinical trials have been performed and are ongoing.
Dental stem cells could potentially repair damaged tooth tissues such as dentin, periodontal ligament, and dental pulp ( Gronthos et al , 2002 ; Ohazama et al , 2004 ; Jo et al , 2007 ; Ikeda et al , 2009 ; Balic et al , 2010 ; Volponi et al , 2010 ). Moreover, as the behavior of dental stem cells is similar to MSCs, dental stem cells could also be used to facilitate the repair of non-dental tissues such as bone and nerves ( Huang et al , 2009 ; Takahashi et al , 2010 ). Several populations of cells with stem cell properties have been isolated from different parts of the tooth. These include cells from the pulp of both exfoliated (children's) and adult teeth, the periodontal ligament that links the tooth root with the bone, the tips of developing roots, and the tissue that surrounds the unerupted tooth (dental follicle) ( Bluteau et al , 2008 ). These cells probably share a common lineage from neural crest cells, and all have generic mesenchymal stem cell-like properties, including expression of marker genes and differentiation into mesenchymal cells in vitro and in vivo ( Bluteau et al , 2008 ). different cell populations do, however, differ in certain aspects of their growth rate in culture, marker gene expression, and cell differentiation. However, the extent to which these differences can be attributed to tissue of origin, function, or culture conditions remains unclear.
There are several issues determining the long-term outcome of stem cell–based therapies, including improvements in the survival, engraftment, proliferation, and regeneration of transplanted cells. The genomic and epigenetic integrity of cell lines that have been manipulated in vitro prior to transplantation play a pivotal role in the survival and clinical benefit of stem cell therapy. Although stem cells possess extensive replicative capacity, immune rejection of donor cells by the host immune system post-transplantation is a primary concern ( Negro et al , 2012 ). Recent studies have shown that the majority of donor cell death occurs in the first hours to days after transplantation, which limits the efficacy and therapeutic potential of stem cell–based therapies ( Robey et al , 2008 ).
Although mouse and human ES cells have traditionally been classified as being immune privileged, a recent study used in vivo , whole-animal, live cell-tracing techniques to demonstrate that human ES cells are rapidly rejected following transplantation into immunocompetent mice ( Swijnenburg et al , 2008 ). Treatment of ES cell-derived vascular progenitor cells with inter-feron γ (to upregulate major histocompatibility complex (MHC) class I expression) or in vivo ablation of natural killer (NK) cells led to enhanced progenitor cell survival after transplantation into a syngeneic murine ischemic hindlimb model. This suggests that MHC class I-dependent, NK cell-mediated elimination is a major determinant of graft survivability ( Ma et al , 2010 ). Given the risk of rejection, it is likely that initial therapeutic attempts using either ES or iPS cells will require adjunctive immunosuppressive therapy. Immunosuppressive therapy, however, puts the patient at risk of infection as well as drug-specific adverse reactions. As such, determining the mechanisms regulating donor graft tolerance by the host will be crucial for advancing the clinical application of stem cell–based therapies.
An alternative strategy to avoid immune rejection could employ so-called ‘gene editing’. Using this technique, the stem cell genome is manipulated ex vivo to correct the underlying genetic defect prior to transplantation. Additionally, stem cell immunologic markers could be manipulated to evade the host immune response. Two recent papers offer alternative methods for ‘gene editing’. Soldner et al (2011) used zinc finger nuclease to correct the genetic defect in iPS cells from patients with Parkinson's disease because of a mutation in the α-Synuclein (α-SYN) gene. Liu et al (2011) used helper-dependent adenoviral vectors (HDAdV) to correct the mutation in the Lamin A (LMNA) gene in iPS cells derived from patients with Hutchinson–Gilford Progeria (HGP), a syndrome of premature aging. Cells from patients with HGP have dysmorphic nuclei and increased levels of progerin protein. The cellular phenotype is especially pronounced in mature, differentiated cells. Using highly efficient helper-dependent adenoviral vectors containing wild-type sequences, they were able to use homologous recombination to correct two different Lamin A mutations. After genetic correction, the diseased cellular phenotype was reversed even after differentiation into mature smooth muscle cells. In addition to the potential therapeutic benefit, ‘gene editing’ could generate appropriate controls for in vitro studies .
Finally, there are multiple safety and toxicity concerns regarding the transplantation, engraftment, and long-term survival of stem cells. Donor stem cells that manage to escape immune rejection may later become oncogenic because of their unlimited capacity to replicate ( Amariglio et al , 2009 ). Thus, ES and iPS cells may need to be directed into a more mature cell type prior to transplantation to minimize this risk. Additionally, generation of ES and iPS cells harboring an inducible ‘kill-switch’ may prevent uncontrolled growth of these cells and/or their derivatives. In two ongoing human trials with ES cells, both companies have provided evidence from animal studies that these cells will not form teratomas. However, this issue has not been thoroughly examined, and enrolled patients will need to be monitored closely for this potentially lethal side effect.
In addition to the previously mentioned technical issues, the use of ES cells raises social and ethical concerns. In the past, these concerns have limited federal funding and thwarted the progress of this very important research. Because funding limitations may be reinstituted in the future, ES cell technology is being less aggressively pursued and young researchers are shying away from the field.
Stem cell technology is unique in that it possesses both diagnostic and therapeutic potential. iPS cells and their derivatives have provided excellent in vitro models of disease processes. Moreover, patient-specific iPS cell models will serve as an invaluable tool for drug discovery and toxicology studies. The therapeutic potential of these cells to regenerate functional tissue and replace damaged tissue has vast potential. Additionally, stem cell–based therapies may enhance the body's own ability to repair itself. As previously mentioned, MSCs exert potent paracrine effects that are thought to be beneficial. For these reasons, the potential of stem cells to cure disease is virtually limitless. Nonetheless, major obstacles to safely implement these therapies remain and must be systematically addressed. Most immediate among these is the identification of stem cell populations that can be maintained and expanded in culture to provide the large numbers needed to be therapeutically useful. Additionally, the potential for cancerous transformation and immuno-logic rejection is largely unexplored. These issues highlight the potential danger of implementing stem cell therapies before they have been adequately studied in vitro and in vivo in animal models. More intensive characterization is required to avoid the possible lethal complications of this type of therapy. Although the current therapeutic potential of stem cell technology is in its infancy, basic research discoveries in the standardization of stem cell derivation, culture, and differentiation techniques, together with improved transplantation, engraftment, and survival strategies, will aid in the development of safer and more effective stem cell–based therapies in the future.
Author contributions
A. Leventhal, G. Chen and A. Negro wrote separate parts of the review. M. Boehm did overall coordination and final review.
- Amado LC, Saliaris AP, Schuleri KH, et al. Cardiac repair with intramyocardial injection of allogeneic mesenchymal stem cells after myocardial infarction. Proc Natl Acad Sci U S A. 2005; 102 :11474–11479. [ PMC free article ] [ PubMed ] [ Google Scholar ]
- Amariglio N, Hirshberg A, Scheithauer BW, et al. Donor-derived brain tumor following neural stem cell transplantation in an ataxia telangiectasia patient. PLoS Med. 2009; 6 :e1000029. [ PMC free article ] [ PubMed ] [ Google Scholar ]
- Artlett CM, Smith JB, Jimenez SA. Identification of fetal DNA and cells in skin lesions from women with systemic sclerosis. N Engl J Med. 1998; 338 :1186–1191. [ PubMed ] [ Google Scholar ]
- Balic A, Aguila HL, Caimano MJ, Francone VP, Mina M. Characterization of stem and progenitor cells in the dental pulp of erupted and unerupted murine molars. Bone. 2010; 46 :1639–1651. [ PMC free article ] [ PubMed ] [ Google Scholar ]
- Bergmann O, Bhardwaj RD, Bernard S, et al. Evidence for cardiomyocyte renewal in humans. Science. 2009; 324 :98–102. [ PMC free article ] [ PubMed ] [ Google Scholar ]
- Bluteau G, Luder HU, De Bari C, Mitsiadis TA. Stem cells for tooth engineering. Eur Cell Mater. 2008; 16 :1–9. [ PubMed ] [ Google Scholar ]
- Broxmeyer HE, Douglas GW, Hangoc G, et al. Human umbilical cord blood as a potential source of transplantable hematopoietic stem/progenitor cells. Proc Natl Acad Sci U S A. 1989; 86 :3828–3832. [ PMC free article ] [ PubMed ] [ Google Scholar ]
- Casper J, Wolff D, Knauf W, et al. Allogeneic hematopoietic stem-cell transplantation in patients with hematologic malignancies after dose-escalated treosulfan/fludarabine conditioning. J Clin Oncol. 2010; 28 :3344–3351. [ PubMed ] [ Google Scholar ]
- Codina M, Elser J, Margulies KB. Current status of stem cell therapy in heart failure. Curr Cardiol Rep. 2010; 12 :199–208. [ PMC free article ] [ PubMed ] [ Google Scholar ]
- Coulombel L. Identification of hematopoietic stem/progenitor cells: strength and drawbacks of functional assays. Oncogene. 2004; 23 :7210–7222. [ PubMed ] [ Google Scholar ]
- Dai W, Hale SL, Martin BJ, et al. Allogeneic mesenchymal stem cell transplantation in postinfarcted rat myocardium: short- and long-term effects. Circulation. 2005; 112 :214–223. [ PubMed ] [ Google Scholar ]
- Daniela F, Vescovi AL, Bottai D. The stem cells as a potential treatment for neurodegeneration. Methods Mol Biol. 2007; 399 :199–213. [ PubMed ] [ Google Scholar ]
- Deans RJ, Moseley AB. Mesenchymal stem cells: biology and potential clinical uses. Exp Hematol. 2000; 28 :875–884. [ PubMed ] [ Google Scholar ]
- Deb A, Wang S, Skelding KA, Miller D, Simper D, Caplice NM. Bone marrow-derived cardiomyocytes are present in adult human heart: a study of gender-miatched bone marrow transplantation patients. Circulation. 2003; 107 :1247–1249. [ PubMed ] [ Google Scholar ]
- Forraz N, Pettengell R, Deglesne PA, McGuckin CP. AC133+ umbilical cord blood progenitors demonstrate rapid self-renewal and low apoptosis. Br J Haematol. 2002; 119 :516–524. [ PubMed ] [ Google Scholar ]
- Gan OI, Murdoch B, Larochelle A, Dick JE. Differential maintenance of primitive human SCID-repopulating cells, clonogenic progenitors, and long-term culture-initiating cells after incubation on human bone marrow stromal cells. Blood. 1997; 90 :641–650. [ PubMed ] [ Google Scholar ]
- Gronthos S, Brahim J, Li W, et al. Stem cell properties of human dental pulp stem cells. J Dent Res. 2002; 81 :531–535. [ PubMed ] [ Google Scholar ]
- Hakuno D, Fukuda K, Makino S, et al. Bone marrow-derived regenerated cardiomyocytes (CMG Cells) express functional adrenergic and muscarinic receptors. Circulation. 2002; 105 :380–386. [ PubMed ] [ Google Scholar ]
- Hill BT, Copelan EA. Acute myeloid leukemia: when to transplant in first complete remission. Curr Hematol Malig Rep. 2010; 5 :101–108. [ PubMed ] [ Google Scholar ]
- Hoff NP, Bruch-Gerharz D. The role of hematopoietic stem-cell transplantation in cutaneous T-cell lymphoma. G Ital Dermatol Venereol. 2010; 145 :345–359. [ PubMed ] [ Google Scholar ]
- Hu BY, Weick JP, Yu J, et al. Neural differentiation of human induced pluripotent stem cells follows developmental principles but with variable potency. Proc Natl Acad Sci U S A. 2010; 107 :4335–4340. [ PMC free article ] [ PubMed ] [ Google Scholar ]
- Huang GT, Gronthos S, Shi S. Mesenchymal stem cells derived from dental tissues vs. those from other sources: their biology and role in regenerative medicine. J Dent Res. 2009; 88 :792–806. [ PMC free article ] [ PubMed ] [ Google Scholar ]
- Humphries A, Graham TA, McDonald SA. Stem cells and inflammation in the intestine. Recent Results Cancer Res. 2011; 185 :51–63. [ PubMed ] [ Google Scholar ]
- Ieda M, Fu JD, Delgado-Olguin P, et al. Direct reprogramming of fibroblasts into functional cardiomyocytes by defined factors. Cell. 2010; 142 :375–386. [ PMC free article ] [ PubMed ] [ Google Scholar ]
- Ikeda E, Morita R, Nakao K, et al. Fully functional bioengineered tooth replacement as an organ replacement therapy. Proc Natl Acad Sci U S A. 2009; 106 :13475–13480. [ PMC free article ] [ PubMed ] [ Google Scholar ]
- Jo YY, Lee HJ, Kook SY, et al. Isolation and characterization of postnatal stem cells from human dental tissues. Tissue Eng. 2007; 13 :767–773. [ PubMed ] [ Google Scholar ]
- Liu GH, Suzuki K, Qu J, et al. Targeted gene correction of laminopathy-associated LMNA mutations in patient-specific iPSCs. Cell Stem Cell. 2011; 8 :688–694. [ PMC free article ] [ PubMed ] [ Google Scholar ]
- Lue J, Lin G, Ning H, Xiong A, Lin CS, Glenn JS. Transdifferentiation of adipose-derived stem cells into hepatocytes: a new approach. Liver Int. 2010; 30 :913–922. [ PubMed ] [ Google Scholar ]
- Ma M, Ding S, Lundqvist A, et al. Major histocompatibility complex-I expression on embryonic stem cell-derived vascular progenitor cells is critical for syngeneic transplant survival. Stem Cells. 2010; 28 :1465–1475. [ PMC free article ] [ PubMed ] [ Google Scholar ]
- Malan D, Friedrichs S, Fleischmann BK, Sasse P. Cardiomyocytes obtained from induced pluripotent stem cells with long-QT syndrome 3 recapitulate typical disease-specific features in vitro. Circ Res. 2011; 109 :841–847. [ PubMed ] [ Google Scholar ]
- Matsuoka S, Tsuji K, Hisakawa H, et al. Generation of definitive hematopoietic stem cells from murine early yolk sac and paraaortic splanchnopleures by aorta-gonad-mesonephros region-derived stromal cells. Blood. 2001; 98 :6–12. [ PubMed ] [ Google Scholar ]
- McGuckin CP, Forraz N. Umbilical cord blood stem cells – an ethical source for regenerative medicine. Med Law. 2008; 27 :147–165. [ PubMed ] [ Google Scholar ]
- McGuckin CP, Pearce D, Forraz N, Tooze JA, Watt SM, Pettengell R. Multiparametric analysis of immature cell populations in umbilical cord blood and bone marrow. Eur J Haematol. 2003; 71 :341–350. [ PubMed ] [ Google Scholar ]
- Meletis J, Terpos E. Transplantation strategies for the management of patients with myelodysplastic syndromes. J Buon. 2009; 14 :551–564. [ PubMed ] [ Google Scholar ]
- Nagaya N, Kangawa K, Itoh T, et al. Transplantation of mesenchymal stem cells improves cardiac function in a rat model of dilated cardiomyopathy. Circulation. 2005; 112 :1128–1135. [ PubMed ] [ Google Scholar ]
- Negro A, St. Hilaire C, Boehm M. Cell-based regenerative therapies: role of major histocompatibility Complex-1 antigen. In: Hayat MA, editor. Stem Cells and Cancer Stem Cells. Vol. 3. Springer; Dordrecht: 2012. pp. 173–178. [ Google Scholar ]
- Ohazama A, Modino SA, Miletich I, Sharpe PT. Stem-cell-based tissue engineering of murine teeth. J Dent Res. 2004; 83 :518–522. [ PubMed ] [ Google Scholar ]
- Park IH, Arora N, Huo H, et al. Disease-specific induced pluripotent stem cells. Cell. 2008; 134 :877–886. [ PMC free article ] [ PubMed ] [ Google Scholar ]
- Pittenger MF, Mackay AM, Beck SC, et al. Multilineage potential of adult human mesenchymal stem cells. Science. 1999; 284 :143–147. [ PubMed ] [ Google Scholar ]
- Portmann-Lanz CB, Schoeberlein A, Portmann R, et al. Turning placenta into brain: placental mesenchymal stem cells differentiate into neurons and oligodendrocytes. Am J Obstet Gynecol. 2010; 202 :294e291–294e211. [ PubMed ] [ Google Scholar ]
- Qiang L, Fujita R, Yamashita T, et al. Directed conversion of Alzheimer's disease patient skin fibroblasts into functional neurons. Cell. 2011; 146 :359–371. [ PMC free article ] [ PubMed ] [ Google Scholar ] Retracted
- Rama P, Matuska S, Paganoni G, Spinelli A, De Luca M, Pellegrini G. Limbal stem-cell therapy and long-term corneal regeneration. N Engl J Med. 2010; 363 :147–155. [ PubMed ] [ Google Scholar ]
- Rinkevich Y, Lindau P, Ueno H, Longaker MT, Weissman IL. Germ-layer and lineage-restricted stem/progenitors regenerate the mouse digit tip. Nature. 2011; 476 :409–413. [ PMC free article ] [ PubMed ] [ Google Scholar ]
- Robey TE, Saiget MK, Reinecke H, Murry CE. Systems approaches to preventing transplanted cell death in cardiac repair. J Mol Cell Cardiol. 2008; 45 :567–581. [ PMC free article ] [ PubMed ] [ Google Scholar ]
- Schachinger V, Erbs S, Elsasser A, et al. Intracoronary bone marrow-derived progenitor cells in acute myocardial infarction. N Engl J Med. 2006; 355 :1210–1221. [ PubMed ] [ Google Scholar ]
- Schneider C, Krause K, Jaquet K, et al. Intramyocardial transplantation of bone marrow-derived stem cells: ultrasonic strain rate imaging in a model of hibernating myocardium. J Card Fail. 2008; 14 :861–872. [ PubMed ] [ Google Scholar ]
- Shimada IS, Spees JL. Stem and progenitor cells for neurological repair: minor issues, major hurdles, and exciting opportunities for paracrine-based therapeutics. J Cell Biochem. 2011; 112 :374–380. [ PMC free article ] [ PubMed ] [ Google Scholar ]
- Soldner F, Laganiere J, Cheng AW, et al. Generation of isogenic pluripotent stem cells differing exclusively at two early onset Parkinson point mutations. Cell. 2011; 146 :318–331. [ PMC free article ] [ PubMed ] [ Google Scholar ]
- Swijnenburg RJ, Schrepfer S, Govaert JA, et al. Immunosuppressive therapy mitigates immunological rejection of human embryonic stem cell xenografts. Proc Natl Acad Sci U S A. 2008; 105 :12991–12996. [ PMC free article ] [ PubMed ] [ Google Scholar ]
- Takahashi K, Yamanaka S. Induction of pluripotent stem cells from mouse embryonic and adult fibroblast cultures by defined factors. Cell. 2006; 126 :663–676. [ PubMed ] [ Google Scholar ]
- Takahashi K, Tanabe K, Ohnuki M, et al. Induction of pluripotent stem cells from adult human fibroblasts by defined factors. Cell. 2007; 131 :861–872. [ PubMed ] [ Google Scholar ]
- Takahashi C, Yoshida H, Komine A, Nakao K, Tsuji T, Tomooka Y. Newly established cell lines from mouse oral epithelium regenerate teeth when combined with dental mesenchyme. In Vitro Cell Dev Biol Anim. 2010; 46 :457–468. [ PMC free article ] [ PubMed ] [ Google Scholar ]
- Terwey TH, Kim TD, Arnold R. Allogeneic hematopoietic stem cell transplantation for adult acute lymphocytic leukemia. Curr Hematol Malig Rep. 2009; 4 :139–147. [ PubMed ] [ Google Scholar ]
- Thomson JA, Itskovitz-Eldor J, Shapiro SS, et al. Embryonic stem cell lines derived from human blastocysts. Science. 1998; 282 :1145–1147. [ PubMed ] [ Google Scholar ]
- Tiscornia G, Monserrat N, Belmonte JC. Modelling long QT syndrome with iPS cells: be still, my beating heart. Circ Res. 2011; 108 :648–649. [ PubMed ] [ Google Scholar ]
- Tse HF, Siu CW, Zhu SG, et al. Paracrine effects of direct intramyocardial implantation of bone marrow derived cells to enhance neovascularization in chronic ischaemic myocardium. Eur J Heart Fail. 2007; 9 :747–753. [ PubMed ] [ Google Scholar ]
- Volponi AA, Pang Y, Sharpe PT. Stem cell-based biological tooth repair and regeneration. Trends Cell Biol. 2010; 20 :715–722. [ PMC free article ] [ PubMed ] [ Google Scholar ]
- de Witte T, Hagemeijer A, Suciu S, et al. Value of allogeneic versus autologous stem cell transplantation and chemotherapy in patients with myelodysplastic syndromes and secondary acute myeloid leukemia. Final results of a prospective randomized European Intergroup Trial. Haematologica. 2010; 95 :1754–1761. [ PMC free article ] [ PubMed ] [ Google Scholar ]
- Yoon YS, Wecker A, Heyd L, et al. Clonally expanded novel multipotent stem cells from human bone marrow regenerate myocardium after myocardial infarction. J Clin Invest. 2005; 115 :326–338. [ PMC free article ] [ PubMed ] [ Google Scholar ]
- Yu J, Vodyanik MA, Smuga-Otto K, et al. Induced pluripotent stem cell lines derived from human somatic cells. Science. 2007; 318 :1917–1920. [ PubMed ] [ Google Scholar ]
- Zhang Q, Shi S, Liu Y, Uyanne J, Shi Y, Le AD. Mesenchymal stem cells derived from human gingiva are capable of immunomodulatory functions and ameliorate inflammation-related tissue destruction in experimental colitis. J Immunol. 2009; 183 :7787–7798. [ PMC free article ] [ PubMed ] [ Google Scholar ]
- Zwi L, Caspi O, Arbel G, et al. Cardiomyocyte differentiation of human induced pluripotent stem cells. Circulation. 2009; 120 :1513–1523. [ PubMed ] [ Google Scholar ]

IMAGES
VIDEO
COMMENTS
In recent years, stem cell therapy has become a very promising and advanced scientific research topic. The development of treatment methods has evoked great expectations. This paper is a review focused on the discovery of different stem cells and the potential therapies based on these cells. The genesis of stem cells is followed by laboratory steps of controlled stem cell culturing and derivation.
Stem cell-based therapy: the history and cell source. a The timeline of major discoveries and advances in basic research and clinical applications of stem cell-based therapy. The term "stem ...
Stem cell-based therapies. Stem cell-based therapies are defined as any treatment for a disease or a medical condition that fundamentally involves the use of any type of viable human stem cells including embryonic stem cells (ESCs), iPSCs and adult stem cells for autologous and allogeneic therapies ().Stem cells offer the perfect solution when there is a need for tissue and organ ...
Another way to differentiate these cells is based on their origins. Stem cells can be extracted from different sources, including bone marrow, amniotic cells, adipose tissue, umbilical cord, and placental tissue. Stem cells began their role in modern regenerative medicine in the 1950's with the first bone marrow transplantation occurring in 1956.
Stem cells have been the focus of hope, hype, and a great deal of research. ... that could be grown in culture long term without loss of stem-cell properties. ... et al. Global position paper on ...
Dusko Ilic and Caroline Ogilvie. The number of clinical trials using human pluripotent stem cells (hPSC)—both embryonic and induced pluripotent stem cells (hESC/iPSC)—has expanded in the last several years beyond expectations. By the end of 2021, a total of 90 trials had been registered in 13 countries with more than 3000 participants.
Abstract. The terms MSC and MSCs have become the preferred acronym to describe a cell and a cell population of multipotential stem/progenitor cells commonly referred to as mesenchymal stem cells ...
The term 'stamzelle or stem cell' was initially used by a German biologist Ernest Haeckel in 1868 for a fertilized egg that develops into a complete organism (Reimer 1868). The term was also used for the single-celled organism that is considered as an ancestor to all the living organisms. The stem cell research actually started with the ...
In recent years, stem cell therapy has become a very promising and advanced scientific research topic. The development of treatment methods has evoked great expectations. This paper is a review focused on the discovery of different stem cells and the potential therapies based on these cells. The genesis of stem cells is followed by laboratory ...
This introductory chapter is designed as a guide to understanding all the new sources and types of stem cells now in clinical trials, as well as the surface markers that help identify each type of cell. One of the most exciting advances in medicine today has been the use of stem cell and gene therapy for repair in both acute and chronic illness.
About the journal. STEM CELLS, a peer-reviewed journal published monthly, provides a forum for prompt publication of original investigative papers and concise reviews.STEM CELLS is read and written by clinical and basic scientists whose expertise encompasses the rapidly expanding fields of stem and progenitor cell biology.. STEM CELLS welcomes original articles and concise reviews describing ...
Regulation of and pathways that govern stem cell self-renewal and/or differentiation. Cellular interactions and signaling pathways necessary for tissue specificity. Molecules and signaling pathways that modulate homing or mobilization of tissue-specific stem/progenitor cells. Functional properties of tissue-specific and progenitor cells.
Stem cells are cells that have the capacity to self-renew by dividing and to develop into more mature, specialised cells. Stem cells can be unipotent, multipotent, pluripotent or totipotent ...
This paper is a review focused on the discovery of different stem cells and the potential therapies based on these cells. ... Stem cells of human exfoliated ... Pirozzi G, Menditti D, De Rosa A, Carinci F, Laino G. Long-term cryopreservation of dental pulp stem cells (SBP-DPSCs) and their differentiated osteoblasts: a cell source for tissue ...
STEM CELLS, a peer reviewed journal published monthly, provides a forum for prompt publication of original investigative papers and concise reviews. STEM CELLS is read and written by clinical and basic scientists whose expertise encompasses the rapidly expanding fields of stem and progenitor cell biology. STEM CELLS welcomes original articles and concise reviews describing basic laboratory ...
Stem cells: The body's master cells. Stem cells are a special type of cells that have two important properties. They are able to make more cells like themselves. That is, they self-renew. And they can become other cells that do different things in a process known as differentiation. Stem cells are found in almost all tissues of the body.
From 1971 to 2021, 40,183 research papers were published regarding stem-cell-based therapies. ... There are several concerns regarding stem-cell-based therapies, including genetic instability after long-term expansion, stem cell migration to inappropriate regions of the body, immunological reaction, and so on. However, all challenges depend on ...
Stem cells are immature tissue precursor cells that can self-renew, create clonal cell populations, and develop into a variety of cell lineages . The therapeutic potential of stem cells can be attributed to several key mechanisms of action including their exceptional homing, differentiation abilities, and their secreted bioactive factors, known ...
Cells is an international peer-reviewed open access semimonthly journal published by MDPI. Please visit the Instructions for Authors page before submitting a manuscript. The Article Processing Charge (APC) for publication in this open access journal is 2700 CHF (Swiss Francs). Submitted papers should be well formatted and use good English.
Cellular & Molecular Immunology - Mesenchymal stem/stromal cells (MSCs): origin, immune regulation, and clinical applications
Call for Papers. STEM CELLS, a peer-reviewed journal published monthly, provides a forum for prompt publication of original investigative papers and concise reviews.STEM CELLS is read and written by clinical and basic scientists whose expertise encompasses the rapidly expanding fields of stem and progenitor cell biology.. Discover the benefits of publishing with us.
Stem cells are defined as precursor cells that have the capacity to self-renew and to generate multiple mature cell types. Only after collecting and culturing tissues is it possible to classify cells according to this operational concept. ... Willenbring H. On the origin of the term 'stem cell'. Cell Stem Cell. 2007; 1:35-38. [Google ...
Mesenchymal stem cells (MSCs) derived from fetal membranes (FMs) have the potential to exhibit immunosuppression, improve blood flow, and increase capillary density during transplantation. In the field of medicine, opening up new avenues for disease treatment.
Objective Peritoneal fibrosis (PF) is the main cause of declining efficiency and ultrafiltration failure of the peritoneum, which restricts the long-term application of peritoneal dialysis (PD). This study aimed to investigate the therapeutic effects and mechanisms of bone marrow mesenchymal stem cells-derived exosomes (BMSC-Exos) on PF in response to PD. Methods Small RNA sequencing analysis ...
Here, we use long-term human stem cell-derived cortical organoids to assess SARS-CoV2 infectivity of brain cells and unravel the cell-type tropism and its downstream pathological effects. Our results show consistent and reproducible low levels of SARS-CoV2 infection of astrocytes, deep projection neurons, upper callosal neurons and inhibitory ...
The fourth main controversy concerns whether adult stem cells are as beneficial as embryonic stem cells. A seminal paper from a group led by ... et al. Hematopoietic reconstitution by multipotent adult progenitor cells: precursors to long-term hematopoietic stem cells. J Exp Med. 2007; 204 (1):129-139. [PMC free article] [Google Scholar ...
The cell suspension was then passed through a 70 µm nylon mesh cell strainer (BD Biosciences, San Jose, CA) to obtain a single-cell suspension. Bone marrow-derived stem cells were enriched in the ...
Organelle-selective vision provides insights into the physiological response of plants and crops to environmental stresses in sustainable agriculture ecosystems. Biological applications often require two-photon excited fluorophores with low phototoxicity, high brightness, deep penetration, and tuneable cell Journal of Materials Chemistry B HOT Papers
A major limitation of chimeric antigen receptor (CAR) T cell therapies is the poor persistence of these cells in vivo 1.The expression of memory-associated genes in CAR T cells is linked to their ...
Two recent papers offer alternative methods for 'gene editing'. Soldner et al ... engraftment, and long-term survival of stem cells. Donor stem cells that manage to escape immune rejection may later become oncogenic because of their unlimited capacity to replicate (Amariglio et al, 2009). Thus, ES and iPS cells may need to be directed into ...